Services on Demand
Journal
Article
Indicators
-
Cited by SciELO
-
Access statistics
Related links
-
Similars in SciELO
Share
Abanico veterinario
On-line version ISSN 2448-6132Print version ISSN 2007-428X
Abanico vet vol.13 Tepic Jan./Dec. 2023 Epub Oct 27, 2023
https://doi.org/10.21929/abavet2023.15
Original article
Evaluation of antiparasitic effect of silver nanoparticles against Trypanosoma cruzi in vitro
1Escuela Superior de Apan, Universidad Autónoma del Estado de Hidalgo, México.
2Doctorado en Nanociencias y Nanotecnología, Centro de Investigación y de Estudios Avanzados del Instituto Politécnico Nacional, México.
3Centro de Investigación y Estudios Avanzados en Salud Animal, Facultad de Medicina Veterinaria y Zootecnia, Universidad Autónoma del Estado de México, México.
4Laboratorio Avanzados de Nanoscopía Electrónica (LANE), Centro de Investigación y de Estudios Avanzadosdel Instituto Politécnico Nacional, México.
American trypanosomiasis is one the globally neglected tropical disease, and is cause by the protozoan haemoflagellate Trypanosoma cruzi (Tc). Currently, the anti-Tc drugs are limited and no vaccine is available. The objective of the study was to determine the effects of silver nanoparticles (AgNPs) against trypomastigotes of the Sylvio X10/4 (TcI) and Esmeraldo (TcII) strains. The AgNPs were obtained by the reduction method and a structural characterization was carried out. The cytotoxic activity of the AgNPs (antiTc activity) was determined by calculating the values of half the maximum inhibitory concentration (IC50) in HaCaT cells and parasites. AgNPs showed no-cytotoxicity against HaCaT cells at the IC50 values assayed in parasites, and the calculated IC50 trypomastigotes was 4.05 µg/ml and 6.16 µg/ml for TcI and TcII strains, respectively. AgNPs were more active against both Trypanosoma cruzi strains than nifurtimox (400 µg/ml) in vitro assays. These results lay the groundwork for further evaluation of AgNPs, to investigate its chemotherapeutic value to figh T. cruzi infection in animal models.
Keywords: Trypanosoma cruzi; Chagas; AgNP; in vitro; silver
La tripanosomiasis Americana es una de las enfermedades tropicales desatendidas a nivel mundial, y es causada por el protozoario hemoflagelado Trypanosoma cruzi (Tc). Actualmente, los fármacos anti-Tc son limitados y no hay vacuna disponible. El objetivo de esta investigación fue evaluar el efecto de las nanopartículas de plata (AgNPs) contra los tripomastigotes de las cepas Sylvio X10/4 (TcI) y Esmeraldo (TcII). Las AgNPs se obtuvieron por el método de reducción y se les realizó una caracterización estructural. La actividad citotóxica de las AgNPs (actividad anti-Tc) se determinó mediante el cálculo de los valores de la mitad de la concentración inhibitoria máxima (IC50) en las células HaCaT y en los parásitos. Las AgNPs no mostraron citotoxicidad contra las células HaCaT en los valores de IC50 probadas en los parásitos, y la IC50 calculada en los tripomastigotes fue de 4.05 µg/ml y de 6.16 µg/ml en las cepas TcI y TcII respectivamente. Las AgNPs fueron más activas contra las dos cepas de Trypanosoma cruzi, que el nifurtimox (400 µg/ml) in vitro. Estos resultados sientan las bases para la evaluación mayor de las AgNPs, por investigar su potencial quimioterapéutico para combatir la infección por T. cruzi en modelos animales.
Palabras claves: Trypanosoma cruzi; Chagas; AgNP; in vitro; plata
INTRODUCTION
Trypanosoma cruzi (T. cruzi) is a flagellated protozoan from the Kinetoplastida order (ElSayed et al., 2005). It is the causative agent of American trypanosomiasis, a vector-borne parasitic disease endemic to South America, Central America and Mexico. T. cruzi represents a major public health problem, with approximately 8 million people infected and 25 million at risk of infection (Ibáñez-Cervantes et al., 2018). In 3-5% of acutely affected patients, infection may result in several conditions: 1) myocarditis, which may progress to heart failure and death; 2) destructive lesions of cardiomyocytes and cardiac nerves, which may also progress to heart failure; or 3) meningoencephalitis (James et al., 2005). In chronically infected patients, the disease may go unnoticed for several years, until 20-35% of these patients develop irreversible lesions of the autonomic nervous system. Chagas disease represents the leading cause of cardiac lesions in young, economically productive adults in endemic countries (El-Sayed et al., 2005; James et al., 2005).
Currently, treatment options are limited and no vaccine is available to prevent or control T. cruzi infection. The drugs approved by WHO for the treatment of Chagas disease, such as nifurtimox (NFMX) and benznidazole (BNZ), have at least four disadvantages (Colantonio et al., 2016). First, due to the harsh side effects of these drugs, about 40% of patients drop out of treatment. Secondly, these drugs work most effectively during the acute phase of the infection; however, as it is difficult to detect, most people start drug treatment until the chronic phase of the disease has been established, with a consequent reduction in the antiparasitic efficacy of the drugs (Colantonio et al., 2016). Third, public access to therapeutic drugs is limited (Chaves et al., 2017). Fourth, some T. cruzi strains are resistant to NFMX and BZN, which makes their efficacy suboptimal (Toledo et al., 2004). These factors emphasize the need to develop better anti‒T. cruzi drugs and/or new treatment strategies for American trypanosomiasis.
Current medical trends include nanomaterials, especially noble metals such as gold and silver. These materials have had biomedical applications since ancient times (Ge et al., 2014). Recent research regarding gold nanoparticles has been oriented to the treatment of cancer, while silver nanoparticles (AgNPs) have been used to fight pathogens (bacteria, fungi, viruses and protozoa) (Ge et al., 2014).
Crystalline silver nanostructures are available in many shapes and sizes (Sun et al., 2002) that can be used as medical treatments (Dankovich et al., 2011; Dubas et al., 2006; Kokura et al., 2010; Lee et al., 2006), but the effect of silver nanoparticles on parasitic protozoa is still incompletely understood (Mao et al., 2016). In this sense, two studies have reported that traditional method to synthesize the AgNPs may have cytotoxic properties against the protozoa pathogens Leishmania tropica and Toxoplasma gondii (Allahverdiyev et al., 2011; Adeyemi et al., 2017) and although recently Brito et al., 2020 biosynthesized AgNPs from corn cobs containing xylans were able of causing death of the Y strain of the T. cruzi.
The aim of the present study was to evaluate the cytotoxic potential of AgNPs on trypomastigotes of two T. cruzi biotypes in vitro. To our knowledge, this is the first attempt to define how the dose and structural characteristics of AgNPs affect T. cruzi cultures in vitro, in an effort to find a safe alternative for the treatment of Chagas disease.
Synthesis of silver nanoparticles
Inorganic silver (AgNO3, ≥ 99.0%), and sodium borohydride (NaBH4, ≥ 99.99%) were purchased from Sigma-Aldrich, USA. All reactions were carried out using deionized water (18MΩ; Millipore). The chosen method induces the dispersion of nanoparticles through a stable colloidal solution, generating a concentration gradient. Sodium borohydride was used as a reducing agent under a cold bath with magnetic stirring (Mulfinger et al., 2007). The AgNP solution (Figure 1) was produced using 0.1 mM AgNO3/50 mL (H2O).
AgNPs prepared in aqueous solution facilitated the study of the interaction between the nanoparticles and the parasite, without the intervention of any surfactant or dispersion medium other than deionized water. This was achieved after several pilot syntheses with two reducing agents (citrate and borohydride). The borohydride produced a more stable and concentrated synthesis (0.1 mM AgNPs) that served to achieve the doses of 1-12 µg used by Allahverdiyev et al., 2011 against Leishmania tropica. Sterility of AgNPs was tested by incubating the nanoparticles in sterile Luria Bertani broth (200 µl/L) at 37ºC for two weeks.
Structural characterization of AgNPs
The nanoparticles were characterized by UV-Vis spectroscopy, transmission electron microscopy (TEM) and high-resolution transmission electron microscopy (HRTEM) using a 1% dilution of AgNPs suspended in deionized water (v/v). A Genesys 10S VIS (Thermo Fisher Scientific, Waltham, Massachusetts, USA) was used to perform UV-Vis spectroscopy and verify the characteristic surface plasmon resonance wavelength (400450 nm) of AgNPs (Mulfinger et al., 2007). Aliquots (40 μL) of AgNPs dispersed in methanol (1:5) were placed on 400 carbon-coated copper grids, dried in a desiccator at 25 °C for 24 h, and then observed in a JEM-ARM200F-TEM at 120 kV. To determine the crystallographic direction of AgNPs facets by fast Fourier transform (FFT), interatomic distances were observed in a JEM-ARM200F-TEM at 200 kV (HRTEM) and analyzed with Garantia Microscopy Suite Digital Micrograph Software.
Parasite culture
Trypomastigotes from the T. cruzi strains Sylvio X10/4 (TcI) and Esmeraldo (TcII) were cultured in monolayers of Vero cells, which were kept in DMEM medium supplemented with 2% fetal bovine serum [FBS] and 1% penicillin‒streptomycin (Thermo Fisher Scientific, Waltham, Massachusetts, USA) under controlled conditions (37°C, 5% CO2, and saturated humidity) (Aparicio-Burgos et al., 2015). As trypomastigotes started bursting out of cells, free parasites were collected and prepared for the AgNP toxicity assay.
Trypomastigotes were placed in 15 mL conical tubes and centrifuged at 704 × g for 7 min. Supernatants were discarded and pellets were re-suspended in 1 mL of fresh DMEM medium. The number of parasites was estimated with a hemocytometer and adjusted to 1×106 per well in a 96-well tissue culture dish for the toxicity assay.
AgNPs toxicity assay for T. cruzi in vitro
Trypomastigotes (1×106 per well) were placed in 96 well plates (Sarstedt, USA) in DMEM supplemented medium and cultured at 37°C, 5% CO2, and saturated humidity, with different AgNP doses (1, 5, 10 or 12 μg/100 μL). The assay was performed by triplicate with untreated (- Ctrl) or NFMX (400 μg/100 μL, Lampit®, Bayer) treated trypomastigotes as negative and positive controls. NFMX was prepared as described by Rolón et al., 2006. Briefly, one tablet of the commercial presentation of NFMX (120 mg) was ground in a sterile mortar and pestle and resuspended in 1 mL of dimethyl sulfoxide (DMSO). The final concentration of DMSO in the culture media never exceeded 0.3% (v/v).
Plates were incubated for 24 hours under the same controlled conditions. After drug treatment, parasite and cell viability were estimated by MTS (3-[4,5,dimethylthiazol-2-yl]5-[3-carboxymethoxyphenyl]-2-[4-sulfophenyl]-2H-tetrazolium, internal salt) of CellTiter 96 kit® Aqueous One Solution (Promega, USA), following the manufacturer's instructions, by colorimetry at 490 nm wavelength. For this assay, optical density (OD) values correlated positively with cell viability. All experiments were performed in triplicate. The half-maximal inhibitory concentration (IC50) for each T. cruzi biotype was calculated from the dose-response curve (Attarde et al., 2020; Barile & Hopkinson, 1993).
In vitro cytotoxic assays in a human keratinocyte cell line (HaCaT)
HaCaT cells (an immortal cell line of non-cancerous human keratinocytes) (2×104/well) were cultured in a 96-well plate (Sarstedt, USA) in supplemented DMEM (2% FBS, penicillin 10,000 units/mL and streptomycin 10,000 µg/mL) and AgNPs at 1, 3, 5, 10 or 12 µg/µL. For each experiment, cells from at least three wells were left untreated (control). The plate was incubated for 48 h under controlled conditions (37 °C, 5 % CO2 and saturated humidity). After treatment, cell viability was estimated by MTS (3[4,5,dimethylthiazol-2-yl]-5-[3-carboxymethylthioxy-phenyl]-2-[4-sulfophenyl]-2Htetrazolium, internal salt) of CellTiter 96 kit® Aqueous One Solution (Promega, USA), following the manufacturer's instructions. The metabolic activity of MTS-treated cells was estimated by colorimetry at a wavelength of 490 nm.
Statistical analyses
ANOVA was used to analyze results from the in vitro viability assay. Mean differences for all assays were assessed using a Tukey test. Differences were considered significant at P < 0.05. Statistical analyses were conducted with the GraphPad Prism 5.0 software package (GraphPad Software Inc., USA).
RESULTS
Characterization of AgNPs
The UV-Vis spectrum of the aqueous silver colloid (Figure 1) showed a strong peak at 396 nm, a typical surface plasmon resonance (SPR) band of AgNPs. The narrow and symmetric absorption peak indicates a homogeneous size distribution of AgNPs. Micrographs of AgNP samples show hemispherical nanoparticles accompanied by some elongated structures of size 52 nm (Figure 2a and 2b). High-resolution transmission electron microscopy (HRTEM) showed that 80% of the particles were decahedrons (Figure 2c) with {111} facets (Figure 2d).
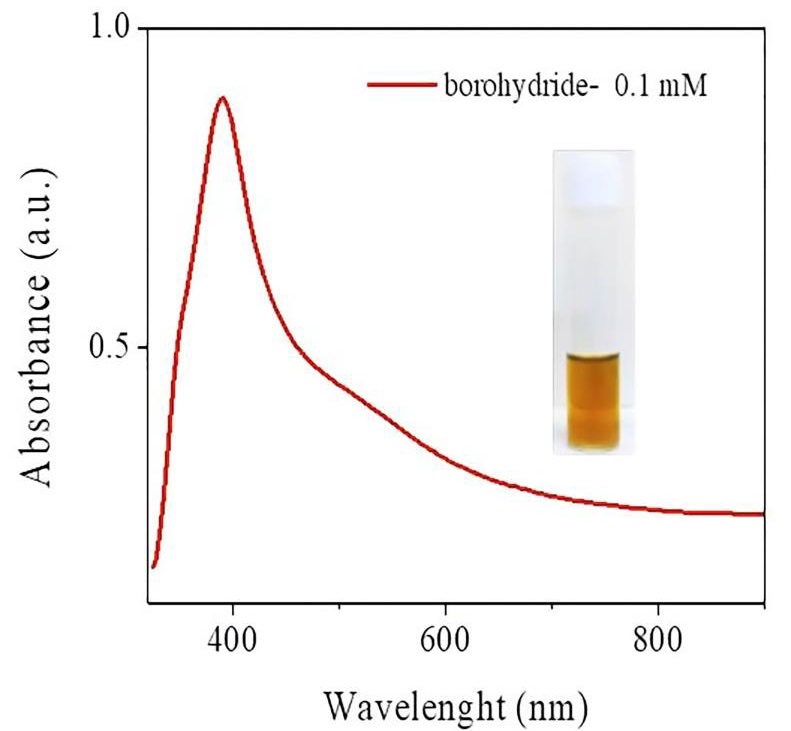
Figure 1 Characterization of AgNPs by UV-Vis Spectroscopy. Absorbance spectra of AgNPs (λmax = 396 nm). The inserted figure displays the photograph of the silver nanoparticles colloid.

Figure 2 Micrographs show silver nanoparticles produced when borohydride was used as a reducing agent at a concentration of 0.1 mM. (a) Transmission Electron Microscopy (TEM) image of silver nanoparticles with an average diameter of 52 nm; (b) Particles are mostly semi-spherical, and some elongated structures are also observed; (c) High Resolution Transmission Electron Microscopy (HRTEM) showed decahedral particles and (d) Fast Fourier Transform (FFT) displayed {111} and {002} facets.
Cell viability for the MTS assay
The trypomastigote viability percentages for both T. cruzi strains showed a dosedependent response; this response was most evident for the Sylvio X10/4 strain, which showed a 50% reduction in parasite viability at the 1 µg dose of AgNPs and a 95% reduction in viability at the highest experimental dose of 12 µg after 24 hours of treatment. However, the Esmeraldo strain showed an 83% reduction in viability at the highest dose (12 µg), showing statistical differences between the Esmeraldo strain and the Sylvio X10/4 strain. Statistical analysis of the results showed differences between the negative control and all other treatments, including the different doses of AgNPs and the positive control (NFMX) (Figure 3).

Figure 3 T. cruzi survival after treatment with any of three different doses of AgNPs (1, 5, 10, 12 µg/100 µl), Nifurtimox (NFMX, 400 µg/100 µl) or without treatment (-Ctrl, used as a negative control for the MTS viability assay). Samples were evaluated after 24 hours of treatment. Each bar represents the absorbance mean value ± SD. Differences (P < 0.05) among groups, according to Tukey’s test, are indicated by characters on top of treatment bars.
IC50 estimation
AgNPs showed no different toxicity between IC50 from the studied strains of T. cruzi. The IC50 for trypomastigotes for Sylvio X10/4 and Esmeraldo strains were 4.05 and 6.16 µg/mL, respectively (Figure 4).

Figure 4 Inhibition of T. cruzi after 24 hours of AgNPs treatment (1, 5, 10, 12 µg/100 µl). Each bar represents the absorbance mean value ± standard deviation (n=6). Within the same time point, statistical differences (P < 0.05) among groups are shown with different characters above the bars acording to Tukey test.
HaCaT cells treated with AgNPs
The viability of HaCaT cells was assessed 48 h after treatment (Figure 5). The toxicity of AgNPs on HaCaT cells was assessed by MTS at different concentrations: 1, 3, 5, 10 or 12 μg/100 μL, which resulted in an IC50 of 10 μg/mL (P<0.05), indicating that AgNPs were approximately 2-fold less toxic to HaCaT cells than trypomastigotes. The results showed that AgNPs were not cytotoxic at the effective dose (IC50 values = 4.05 and 6.16 µg/mL) against TcI and TcII strains of T. cruzi.
DISCUSSION
Chagas disease is a vector-borne disease caused by T. cruzi, a hemoflagellate parasite that often causes debilitating and fatal heart disease. BNZ and NFMX are the only available drugs approved for medical treatment (Chaves et al., 2017; Toledo et al., 2004). However, these drugs induce harsh side effects that prevent patients from concluding treatment. In many cases, T. cruzi is resistant to these drugs, making them unable to eliminate the parasite from infected tissue (Colantino et al., 2016; Toledo et al., 2004). Therefore, public health programs require the development of more effective and less toxic therapeutic drugs for the treatment of Chagas disease.
Silver nanoparticles (AgNPs) have antimicrobial effects not only against fungi (Soliman et al., 2018; Mousavi et al., 2015), Gram-positive (Adibhesami et al., 2017) and Gramnegative bacteria (Pal et al., 2007), but also against protozoa such as Apicomplexa (Toxoplasma gondii) (Adeyemi et al., 2017) and Trypanosomatida (Leishmania tropica and Leishmania infantum) (Baiocco et al., 2011). Here we report the in vitro trypanolytic effects of AgNPs against two different strains of T. cruzi.
For this study, we produced hemispherical, decahedral AgNPs with a mean diameter of 52 nm (Figures 1 and 2), and used them at 5 different doses (1, 3, 5, 10 or 12 μg/mL) to test toxicity in HaCaT cells and trypanolytic activity in trypomastigotes produced in coculture with Vero cell monolayers. We found that silver nanoparticles significantly reduce cell viability in a dose-dependent manner in HaCaT cells. This observation is in agreement with other studies (Chen et al., 2019; Perde-Schrepler et al., 2019). Importantly, AgNPs are not cytotoxic at doses effective against T. cruzi strains, and the toxicity of AgNPs on trypomastigotes was indirectly assessed by an MTS proliferation assay, which showed a negative effect of AgNPs on trypomastigote viability. Figure 3 shows that nanoparticles significantly reduced cell viability compared to the negative control, and that AgNPs had similar trypanolytic power to NFMX at different doses. Although no significant differences in T. cruzi viability were observed with different doses of AgNPs, a dose-dependent trend in viability was observed. These data suggest that higher doses of AgNPs may have additional effects on the viability of T. cruzi trypomastigotes. Interestingly, a relatively low dose (12 μg/100 μL) of AgNPs killed up to 96.3% (with an IC50 = 4.05 - 6.16 µg/100 μL) of trypomastigotes in vitro, whereas NFMX (400 μg/100 μL) killed only 70% (Figures 3 and 4). This also suggests that AgNPs could be a more efficient trypanolytic agent in vitro than NFMX, as observed in the present study, but also in comparison to BNZ nanoparticles (with an IC50 = 36 µg/mL) (Scalise et al., 2016).
T. cruzi has already developed some resistance to BNZ and NFMX, the only clinically approved drugs against Chagas disease (Mejia et al., 2012; Molina et al., 2000). Different T. cruzi biotypes have differential resistance to BNZ. TcI strains of T. cruzi are more resistant to BNZ, whereas TcII strains are more sensitive to this drug (Toledo et al., 2004; Mejia et al., 2012). Here, we found no differences in sensitivity to AgNPs between the two T. cruzi strains tested (Sylvio X10/4 and Esmeraldo) belonging to different biotypes (TcI and TcII, respectively). Although further studies are needed, our data regarding the broad spectrum of antiparasitic activity of AgNPs suggest that nanoparticles could be an interesting option to solve the problem of parasite resistance to other drugs, either used as an alternative drug or in combination with those currently in use.
Further studies should address the effects of AgNPs as alternative drugs against T. cruzi, especially to understand whether these nanoparticles can exert a detrimental effect on amastigotes, the intracellular developmental stage of the parasite in mammals. Leishmania tropica amastigotes have been reported to be more sensitive to AgNPs than promastigotes at a dose of 10 μg/0.1 mL (Allahverdiyev et al., 2011). If that hypothesis holds for T. cruzi, the use of AgNPs in vivo for therapeutic use against Chagas disease would become an interesting topic of study.
AgNPs possess unique physical and electronic properties, which make them excellent candidates for medical applications. The antimicrobial mechanism of AgNPs seems to be related to the formation of free radicals that damage the microorganisms’ membrane (Kim et al., 2007). These properties depend on size, shape, and nanoparticle coating (Dubey et al., 2015). In the present study, we synthesized AgNPs with an average diameter of 52 nm; most of them were hemispherical (decahedral), had {111} and {110} facets, and had acceptable trypanolytic activity. However, we believe that the trypanolytic activity of AgNPs could be improved, if the shape and size of the nanoparticles were adjusted to triangles with a size of 1-10 nm. This type of nanoparticles shows higher antibacterial activity than larger AgNPs with hemispherical shapes due to the higher density on the facets {111}. This allows triangular nanoparticles to freely permeate the plasma membrane and thus induce more damage to microorganisms. In addition, truncated triangular AgNPs require lower doses (1-10 μg/0.1 μL) to exert bactericidal properties than larger spherical and nanorodal AgNPs (12.5 and 50-100 μg/0.1 μL, respectively) (Ge et al., 2014; Dubey et al., 2015; Morones et al., 2005).
CONCLUSIONS
A dose of 12 µg/100 µL dose of AgNPs with an average diameter of 52 nm was cytotoxic for trypomastigotes from TcI and TcII T. cruzi biotypes in vitro. Future studies test triangular shaped AgNPs with {111} facets to improve the antiprotozoal effects of AgNPs on different biotypes of T. cruzi.
Conflict of interest statement
We declare that we have no conflict of interest.
ACKNOWLEDGMENTS
This work was supported by the following grants: UAEH-PTC-714, DSA/103.5/15/10450; UAEH-PTC-673, DSA/103.5/15/7001 and Project for the integration of thematic networks of academic collaboration 2015 "Nanotechnology as a tool for the diagnosis and treatment of infectious diseases" of the academic body UAEM-CA-2015 of the Ministry of Public Education, Mexico.
REFERENCES
Adeyemi OS, Murata Y, Sugi T, Kato K. 2017. Inorganic nanoparticles kill Toxoplasma gondii via changes in redox status and mitochondrial membrane potential. International Journal of Nanomedicine. 12: 1647-1661. ISSN: 1176-9114. https://doi.org/10.2147/IJN.S122178 [ Links ]
Adibhesami M, Ahmadi M, Farshid AA, Sarrafzadeh-Rezaei F, Dalir-Naghadeh B. 2017. Effects of silver nanoparticles on Staphylococcus aureus contaminated open wounds healing in mice: An experimental study. Veterinary Research Forum. 8(1): 23-28. ISSN: 2008-8140. https://www.ncbi.nlm.nih.gov/pmc/articles/PMC5413307/ [ Links ]
Allahverdiyev AM, Abamor ES, Bagirova M, Ustundag CB, Kaya C, Kaya F. Rafailovich M. 2011. Antileishmanial effect of silver nanoparticles and their enhanced antiparasitic activity under ultraviolet light. International Journal of Nanomedicine. 6:2705. ISSN: 1176-9114. https://doi.org/10.2147/IJN.S23883 [ Links ]
Aparicio-Burgos JE, Zepeda-Escobar, JA, de Oca-Jimenez RM, Estrada-Franco JG, Barbabosa-Pliego A, Ochoa-García L, Alejandre-Aguilar R, Rivas N, Peñuelas Rivas G, Val-Arreola M, Shivali Gupta Salazar-García F, Garg NJ, Vázquez-Chagoyán JC. 2015. Immune protection against Trypanosoma cruzi induced by TcVac4 in a canine model. PLoS Neglected Tropical Diseases. 9(4): e0003625. ISSN: 1935-2735. https://doi.org/10.1371/journal.pntd.0003625 [ Links ]
Attarde SS, Pandit, SV. 2020. Anticancer potential of nanogold conjugated toxin GNPNN-32 from Naja naja venom. Journal of Venomous Animals and Toxins including Tropical Diseases. 26. ISSN: 1678-9199. https://doi.org/10.1590/1678-9199-JVATITD2019-0047 [ Links ]
Baiocco P, Ilari A, Ceci, P, Orsini, S, Gramiccia, M, Muccio TD, Colotti G. 2011. Inhibitory effect of silver nanoparticles on trypanothione reductase activity and Leishmania infantum proliferation. ACS Medicinal Chemistry Letters. 2(3): 230-233. ISSN: 1948-5875. https://pubs.acs.org/doi/10.1021/ml1002629 [ Links ]
Barile FA, Arjun, S, Hopkinson, D. 1993. In vitro cytotoxicity testing: biological and statistical significance. Toxicology In Vitro. 7(2):111-116. ISSN: 0887-2333. https://doi.org/10.1016/0887-2333(93)90120-T [ Links ]
Brito TK, Viana, RLS, Moreno CJG, da Silva Barbosa J, de Sousa, JFL, de Medeiros MJC, Rocha HAO. 2020. Synthesis of silver nanoparticle employing corn cob xylan as a reducing agent with anti-Trypanosoma cruzi activity. International Journal of Nanomedicine. 15: 965-979. ISSN: 1176-9114. https://doi.org/10.2147/IJN.S216386 [ Links ]
Chaves GC, Arrieche MAS, Rode J, Mechali D, Reis PO, Stobbaerts A, Girón AE, Nora RI. 2017. Estimating demand for anti-Chagas drugs: a contribution for access in Latin America/Estimacion de la demanda de medicamentos antichagasicos: una contribucion para el acceso en America Latina/Estimativa da demanda de medicamentos antichagasicos: uma contribuicao para o acesso na America Latina. Revista Panamericana de Salud Pública. 41(8):1-8. ISSN: 1680 5348. https://www.scielosp.org/pdf/rpsp/2017.v41/e45/es [ Links ]
Chen L, Wu M, Jiang S, Zhang Y, Li R, Yongbo L, Lin L, Gang W, Ying L, Liming X, Liming X. 2019. Skin toxicity assessment of silver nanoparticles in a 3D epidermal model compared to 2D keratinocytes. International Journal of Nanomedicine. 14:9707-9719. ISSN: 1176-9114. https://doi.org/10.2147/IJN.S225451 [ Links ]
Colantonio LD, Prado N, Segura EL, Sosa ES. 2016. Electrocardiographic abnormalities and treatment with benznidazole among children with chronic infection by Trypanosoma cruzi: a retrospective cohort study. PLoS Neglected Tropical Diseases. 10(5): e0004651. ISSN: 1935-2735. https://doi.org/10.1371/journal.pntd.0004651 [ Links ]
Dankovich TA, Gray DG. 2011. Bactericidal paper impregnated with silver nanoparticles for point-of-use water treatment. Environmental Science & Technology. 45(5): 1992-1998. ISSN: 1520-5851. https://doi.org/10.1021/es103302t [ Links ]
Dubas ST, Kumlangdudsana P, Potiyaraj P. 2006. Layer-by-layer deposition of antimicrobial silver nanoparticles on textile fibers. Colloids and Surfaces A: Physicochemical and Engineering Aspects. 289(1-3): 105-109. ISSN: 0927-7757. https://doi.org/10.1016/j.colsurfa.2006.04.012 [ Links ]
Dubey P, Matai I, Kumar SU, Sachdev A, Bhushan B, Gopinath P. 2015. Perturbation of cellular mechanistic system by silver nanoparticle toxicity: Cytotoxic, genotoxic and epigenetic potentials. Advances in Colloid and Interface Science. 221: 4-21. ISSN: 00018686. https://doi.org/10.1016/j.cis.2015.02.007 [ Links ]
El-Sayed NM, Myler PJ, Bartholomeu DC, Nilsson D, Aggarwal G, Tran AN, Ghedin E, Worthey EA, Delcher AL, Blandin G, Westenberger GS, Caler E, Cerqueira GC, Branche C, Haas B, Anupama A, Arner E, Aslund L, Attipoe P, Bontempi E, Bringaud F, Burton P, Cadag E, Campbell DA, Carrington M, Crabtree J, Darban H, da Silveira JF, de Jong P, Edwards K, Englund PT, Fazelina G, Feldblyum T, Ferella M, Frasch AC, Gull K, Horn D, Hou L, Huang Y, Kindlund E, Klingbeil M, Kluge S, Koo H. 2005. The genome sequence of Trypanosoma cruzi, etiologic agent of Chagas disease. Science. 309(5733): 409-415. ISSN: 00368075. https://doi.org/10.1126/science.1112631 [ Links ]
Ge L, Li Q, Wang M, Ouyang J, Li X, Xing MMQ 2014. Nanosilver particles in medical applications: synthesis, performance, and toxicity. International Journal of Nanomedicine. 9: 2399-2407. ISSN: 1176-9114. https://doi.org/10.2147/IJN.S55015 [ Links ]
Ibáñez-Cervantes G, León-García G, Castro-Escarpulli G, Mancilla-Ramírez J, Victoria-Acosta G, Cureño-Díaz MA, Sosa-Hernández O, Bello-López JM. 2018. Evolution of incidence and geographical distribution of Chagas disease in Mexico during a decade (2007-2016). Epidemiology & Infection. 147:1-7. ISSN: 1469-4409. https://doi.org/10.1017/S0950268818002984 [ Links ]
James TN, Rossi MA, Yamamoto S. 2005. Postmortem studies of the intertruncal plexus and cardiac conduction system from patients with Chagas disease who died suddenly. Progress in Cardiovascular Diseases. 47(4): 258-275. ISSN: 0033-0620. https://doi.org/10.1016/j.pcad.2005.01.003 [ Links ]
Kim JS, Kuk E, Yu KN, Kim JH, Park SJ, Lee HJ, Kim SH, Park YK, Park YH, Hwang CY, Kim YK, Lee YS, Jeong DH, Cho MH. 2007. Antimicrobial effects of silver nanoparticles. Nanomedicine: Nanotechnology, Biology, and Medicine. 3(1): 95-101. ISSN: 1549-9634. https://doi.org/10.1016/j.nano.2006.12.001 [ Links ]
Kokura S, Handa O, Takagi T, Ishikawa T, Naito Y, Yoshikawa T. 2010. Silver nanoparticles as a safe preservative for use in cosmetics. Nanomedicine: Nanotechnology, Biology and Medicine. 6(4): 570-574. ISSN: 1549-9634. https://doi.org/10.1016/j.nano.2009.12.002 [ Links ]
Lee KS, El-Sayed MA. 2006. Gold and silver nanoparticles in sensing and imaging: sensitivity of plasmon response to size, shape, and metal composition. Journal of Physical Chemistry B. 110(39): 19220-19225. ISSN: 1520-5207. https://doi.org/10.1021/jp062536y [ Links ]
Mao BH, Tsai JC, Chen CW, Yan SJ, Wang YJ. 2016. Mechanisms of silver nanoparticleinduced toxicity and important role of autophagy. Nanotoxicology. 10(8): 1021-1040. ISSN: 1743-5404. https://doi.org/10.1080/17435390.2016.1189614 [ Links ]
Mejia AM, Hall BS, Taylor MC, Gómez-Palacio A, Wilkinson SR, Triana-Chávez O, Kelly JM. 2012. Benznidazole-resistance in Trypanosoma cruzi is a readily acquired trait that can arise independently in a single population. The Journal of Infectious Diseases. 206(2): 220-228. ISSN: 1537-6613. https://doi.org/10.1093/infdis/jis331 [ Links ]
Molina J, Martins-Filho O, Brener Z, Romanha AJ, Loebenberg D, Urbina JA. 2000. Activities of the triazole derivative SCH 56592 (posaconazole) against drug-resistant strains of the protozoan parasite Trypanosoma (Schizotrypanum) cruzi in immunocompetent and immunosuppressed murine hosts. Antimicrobial Agents and Chemotherapy. 44(1): 150-155. ISSN: 1098-6596. https://doi.org/10.1128/AAC.44.1.150-155.2000 [ Links ]
Morones JR, Elechiguerra JL, Camacho A, Holt K, Kouri JB, Ramírez JT, Yacaman JM. 2005. The bactericidal effect of silver nanoparticles. Nanotechnology. 16(10): 2346. ISSN: 09574484. https://doi.org/10.1088/0957-4484/16/10/059 [ Links ]
Mousavi SAA, Salari S, Hadizadeh S. 2015. Evaluation of antifungal effect of silver nanoparticles against Microsporum canis, Trichophyton mentagrophytes and Microsporum gypseum. Iran Journal of Biotechnology. 13(4): 38-42. ISSN: 1728-3043. https://doi.org/10.15171/ijb.1302 [ Links ]
Mulfinger L, Solomon, SD, Bahadory M, Jeyarajasingam AV, Rutkowsky SA, Boritz C. 2007. Synthesis and study of silver nanoparticles. Journal of Chemical Education. 84(2): 322. ISSN: 00219584. https://doi.org/10.1021/ed084p322 [ Links ]
Pal S, Tak YK, Song JM. 2007. Does the antibacterial activity of silver nanoparticles depend on the shape of the nanoparticle? A study of the gram-negative bacterium Escherichia coli. Applied and Environmental Microbiology. 73(6):1712-1720. ISSN: 10985336. https://doi.org/10.1128/AEM.02218-06 [ Links ]
Perde-Schrepler M, Florea A, Brie I, Virag P, Fischer-Fodor E, Vâlcan A, Gurzău E, Lisencu C, Maniu A. 2019. Size-dependent cytotoxicity and genotoxicity of silver nanoparticles in cochlear cells in vitro. Journal of Nanomaterials. e6090259. ISSN: 16874129. https://doi.org/10.1155/2019/6090259 [ Links ]
Rolón M, Vega C, Escario JA, Gómez BA. 2006. Development of resazurin microtiter assay for drug sensibility testing of Trypanosoma cruzi epimastigotes. Parasitology Research. 99(2): 103-107. ISSN: 1432-1955. https://doi.org/10.1007/s00436-006-0126-y [ Links ]
Scalise ML, Arrúa EC, Rial MS, Esteva MI, Salomon CJ, Fichera LE. 2016. Promising efficacy of benznidazole nanoparticles in acute Trypanosoma cruzi murine model: in-vitro and in-vivo studies. The American Journal of Tropical Medicine and Hygiene. 95(2): 388393. ISSN: 0002-9637. https://doi.org/10.4269/ajtmh.15-0889 [ Links ]
Soliman H, Elsayed A, Dyaa A. 2018. Antimicrobial activity of silver nanoparticles biosynthesised by Rhodotorula sp. strain ATL72. Egyptian Journal of Basic and Applied Sciences. 5(3): 228-233. ISSN: 2314-808X. https://doi.org/10.1016/j.ejbas.2018.05.005 [ Links ]
Sun Y, Xia Y. 2002. Shape-controlled synthesis of gold and silver nanoparticles. Science. 298(5601): 2176-2179. ISSN: 00368075. https://doi.org/10.1126/science.1077229 [ Links ]
Toledo MJO, Bahia MT, Veloso VM, Carneiro CM, Machado-Coelho GLL, Alves CF, Martins HR, Cruz RE, Tafuri WL, Lana M. 2004. Effects of specific treatment on parasitological and histopathological parameters in mice infected with different Trypanosoma cruzi clonal genotypes. The Journal of Antimicrobial Chemotherapy. 53(6):1045-1053. ISSN: 03057453. https://doi.org/10.1093/jac/dkh224 [ Links ]
Received: August 18, 2022; Accepted: May 31, 2023