Introduction
Silver nanoparticles (Ag NPs) have been extensively studied because of their unique optical, magnetic, electrical, and catalytic properties, with applications in pharmacology, medicine, food processing, and agriculture (Luo, Zhang, Zeng, Zeng, & Wang, 2005; Petica, Gavriliu, Lungu, Buruntea, & Panzaru, 2008; Sondi & Salopek-Sondi, 2004). The synthesis of Ag NPs can be achieved by several methods, including chemical reduction, electrochemical reduction, UV-radiation, photochemical reduction, and ultrasonic assisted, microwave, and laser ablation (Kumar, Smita, Cumbal, & Debut, 2017; Mahendran & Ranjitha-Kumari, 2016). These processes usually have a high cost and involve use of organic solvents and toxic reducing agents such as sodium borohydride and N,N-dimethyl formamide (Raja, Ramesh, & Thivaharan, 2017).
On the other hand, biological synthesis has emerged as a new option for obtaining nanoscale materials, primarily as a result of the increasing need to use environmentally-friendly synthetic methods. Biosynthesis involves the use of microorganisms (bacteria, yeasts, fungi) or plant extracts to achieve the reduction of metal ions, including silver, gold and copper (Basavaraja, Balaji, Lagashetty, Rajasab, & Venkataraman, 2008; Bradley, Schmid, Shevchenko, & Weller, 2004; He et al., 2007; Sastry, Ahmad, Khan, & Kumar, 2003; Vigneshwaran et al., 2007).
Of particular interest herein is that the use of plant extracts acting as reducing agents may provide a quick way of preparing nanoparticles. Examples include the synthesis of metal nanoparticles using as reducing or passivating agents extracts of coriander (Narayanan & Sakthivel, 2008), lemon verbena (Cruz et al., 2010), orange peel (Kaviya, Santhanalakshmi, Viswanathan, Muthumary, & Srinivasan, 2011), or banana peel (Bankar, Joshi, Kumar, & Zinjarde, 2010).
Crataegus spp., a Mexican plant commonly known as tejocote, has medicinal, industrial and ornamental uses (Nieto-Ángel, Pérez-Ortega, Núñez-Colín, Martínez-Solís, & González-Andrés, 2009). The Crataegus gracilior plant extract contains a high percentage of pectin, which is composed mainly of D-galacturonic acid and, in less proportion, D-galactose, L-arabinose, D-xylose and L-rhamnose. In Ag NP synthesis, pectin forms polymer networks in which silver nanoparticles are embedded, also acting as a reducing agent and silver ion protector (Krivorotova et al., 2016; Zahran, Ahmed, & El-Rafie, 2014; Zainudin, Wong, & Hamdan, 2018). To our knowledge, however, this has never been addressed in the literature. Therefore, the objective of the present study was to synthesize Ag NPs using aqueous Crataegus gracilior bark extract as precursor.
Materials and methods
Ag NPs were synthesized using silver nitrate (AgNO3, > 99.8 %, Sigma-Aldrich®, USA) as precursor and aqueous tejocote bark extracts as reducing and passivating agent. Deionized water (18 MΩ·cm-1; Easypure II, Thermo ScientificTM, Spain) was used throughout the experiments and sodium hydroxide (NaOH) was used to adjust the pH.
For the synthesis, 100 mL of aqueous tejocote bark extract were placed in a round-bottomed flask, where the pH was adjusted with 0.1M HCl and 0.1 M NaOH. Then, the solution was heated to 75 °C under constant stirring. When the desired temperature was reached, 10 mL of 0.1 M AgNO3 were added. The final mixture was stirred for 30 min at the same temperature. Preliminary analyses were conducted at solid concentrations of 1, 5, and 10 % (w/v) and pH0 6, 8, and 10. Samples with a 10 % solid concentration and pH 10 contained the smallest and most stable nanoparticles.
Characterization of nanoparticles
Ultraviolet-visible spectroscopy (UV-Vis). Optical absorption spectra for Ag NP suspensions were obtained using a Cary 50 spectrophotometer (Varian®, USA). Ag NP suspensions were diluted at a 5:1 ratio with deionized water (18 μΩ·cm-1) prior to analysis. Deionized water was used as a reference.
Fourier-transform infrared spectroscopy (FTIR). The Ag NP suspension was centrifuged at 13 000 rpm (Sigma 2-16, Sigma Laborzentrifugen GmbH®, Germany) and subsequently dried at room temperature. Dried powdered plant material and Ag NPs were separately mixed with KBr at a 1:10 ratio to form pellets. The spectra were recorded using a spectrometer (Spectrum One, Perkin Elmer®, USA), with a 4 000 to 400 cm-1 frequency range.
Transmission Electron Microscopy (TEM). Ag NPs were examined in a JEM-2010 microscope (JEOL®, Japan) operating at 200 kV and 115 μA, for which a single drop of the colloidal solution was placed on a 200-mesh carbon-coated copper grid (Electron Microscopy Sciences, USA). Additionally, the selected area electron diffraction (SAED) was obtained at a wavelength of 0.0025 nm and a camera length of 20 cm. Average size and size distribution values were obtained by analyzing 150 particles, using PhotoImpact 11 software (Ulead Systems®, USA).
X-ray diffraction (XRD). It was performed in a D8 Advance diffractometer (Bruker®, Germany). The patterns were recorded by CuKα (1.54 A°) with a cupper monochromator. The scanning of Ag NPs was done in a region of 2θ from 5 to 90° at 0.05°/10 s.
Determination of zeta potential and size distribution. The hydrodynamic diameter and polydispersity index of the nanoparticles were determined by dynamic light scattering (DLS) and zeta potential (ZS ZEN3600, Malvern Instruments®, UK). Measurements were performed in triplicate, with each measurement conducted using 1 mL of suspension at room temperature (25 °C). The calculations of electrophoretic mobility were automatically converted into zeta potential values, based on the Smoluchowski model; ten readings were obtained to calculate the average electrical charge.
Results and discussion
UV-Vis. After the addition of AgNO3 to the extract solution, the color of the solution changed from colorless to brown indicating the formation of silver nanoparticles. It is highly possible that pectin, hydrolysable tannins, polyphenols and flavonoids present in the tejocote extract have acted as reducing agents to produce Ag0. Maximum absorbance values for Ag NP with tecojote extracts suspensions were obtained (10 % w/v) at 500 nm.
The position and shape of the absorption band for a surface plasmon depend on particle size and shape: increases in size cause the absorption band to shift towards higher wavelengths (Slistan-Grijalva et al., 2008). Based on the spectral signals obtained (Mitra & Bhaumik, 2007), nanoparticles obtained with the tejocote extracts presented an average size ≤ 30 nm (Figure 1).
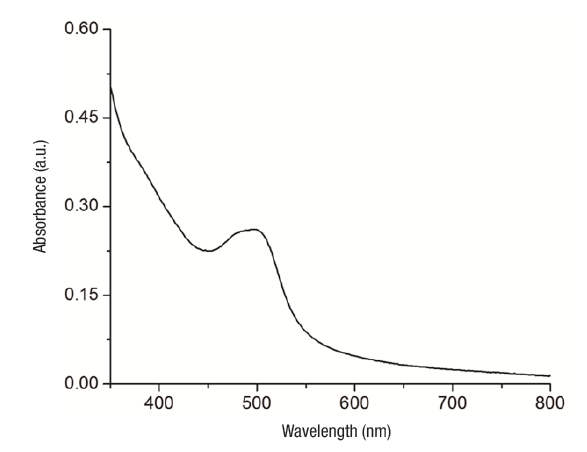
Figure 1 UV-Vis absorption spectrum of a colloidal system of silver nanoparticles with aqueous tejocote (Crataegus gracilior) bark extract.
FTIR analysis. Shown in Figure 2 are the FTIR spectra of the precursors (AgNO3 and tejocote extract) and Ag NPs. The spectrum of tejocote extract denotes absorption bands at 1 045 cm-1 and 1 115 cm-1 (corresponding to CO vibrations), 3 417 cm-1 (amide A, NH stretching and OH bending and stretching), 1 619 cm-1 (amide I, C=O stretching), 1 520 cm-1 (amide II, bending vibrations of NH and CN stretching), and 1 254 cm-1 (amide III, bending vibrations of NH (Hayashi & Mukamel, 2008). FTIR spectra for AgNO3 revealed an intense absorption band at 1 376 cm-1, characteristic of the Ag+NO3 - ion pair.

Figure 2 FTIR spectra of AgNO3, tejocote extract and silver nanoparticles, the last of which interact with different functional groups of carbohydrates and proteins.
On the other hand, in the spectrum of the silver nanoparticles, the absorption bands corresponding to the amide groups shifted to lower wavenumber values. These changes on the position bands may be due to the interactions of proteins that are possibly bound to Ag NPs through the amine groups. Additionally, the band intensity of the ion pair Ag+NO3 - decreased and shifted to a higher wavenumber value. This peak, centered at 1 385 cm-1, is characteristic of the NO3 - ion in free form, and the absorption band displacement is caused by a change in the electronic environment of the anion, as a result of the separation of its counterpart Ag+ (Cho & So, 2006).
TEM. Figure 3a shows the Ag NPs embedded in a polymer network at tejocote concentrations of 10 %. Dark areas corresponded to Ag NPs and light areas to different compounds present in the plant extract. Ag NPs showed an average diameter of 30 nm and a spherical morphology, although the magnification showed an ovoid shape. Figure 3b exemplifies an electron diffraction pattern of the Ag NPs. High magnification evidenced a face-centered cubic (FCC) structure, a spatial group of Fm-3m (225), and a lattice parameter a = 4.09 Å.
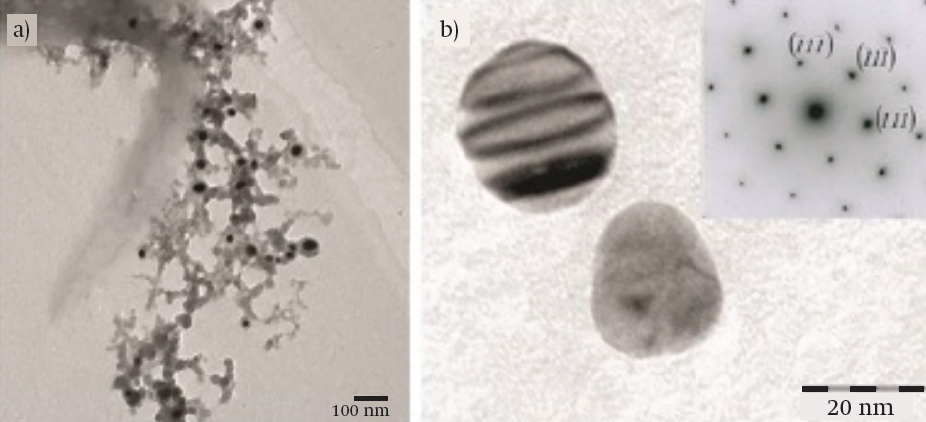
Figure 3 Transmission electron microscopy images of silver nanoparticles (Ag NPs): (a) Ag NPs embedded in a polymer network (10 Kv) and (b) magnified image and electron diffraction pattern of Ag NPs (120 kV). Extinction contour lines shown in the top section of the particle confirmed a variable thickness.
XRD. Results obtained by TEM and calculations using the Scherrer equation were best explained by an average size of 26 nm. Figure 4 shows the X-ray diffraction pattern obtained for Ag NPs. Five Bragg reflections are observed in the diffractogram at 2θ angles centered at 38.0°, 44.0° 64.4°, 77.32°, and 81.34° corresponding to planes (111), (200), (220), (311), and (222) respectively, from the FCC of the Ag with spatial group Fm-3m (225), according to the diffraction analysis PDF-04-0783. The diffractogram indicates the presence of nanometric-size crystals without preferential direction for growth.
Nanoparticle size distribution and zeta potential. According to the DLS analysis, the particles obtained are a polydisperse mixture with a mean hydrodynamic diameter of 108 nm and a polydispersity index of 0.24. On the other hand, the colloidal solution of Ag NPs had a zeta potential value of -21.9 ± 5.11 mV, indicating good NP stability. This stability mainly depends on the surface charge; hence particles with a similar surface charge repel each other due to electrostatic repulsion forces (Khan, Mukherjee, & Chandrasekaran, 2011). According to Sun et al. (2014), a suspension with an absolute zeta potential lower than 20 mV is considered unstable, and its constituent particles will tend to precipitate, whereas an absolute zeta potential greater than 20 mV is indicative of a stable solution.
Conclusions
Biosynthesis of Ag NPs was performed using AgNO3 and aqueous tejocote (Crataegus gracilior) bark extracts as precursors, achieving an efficient, low-cost and environmentally-friendly synthesis. FTIR results confirmed the stabilization of Ag NPs by interacting with organic components in the extract, primarily carbohydrates and proteins. TEM showed that Ag NPs were mostly spherical, with an average size of 30 nm. Additionally, electron diffraction patterns confirmed an FCC structure.