Introduction
Worldwide, 30 % of all medical consultations for musculoskeletal issues are related to tendon injuries, with 4 million new cases reported each year, indicating that it is a frequent health concern. The tendon is an essential dynamic stabilizing element in the human body, enabling the transmission of force generated by muscles to the bone segment, allowing movement to occur. Additionally, it absorbs external forces to reduce muscle overload and prevent injuries1.
Tendinopathy is a common tendon injury with social and economic repercussions in the population. The tendon can undergo an acute pathological process named tendinitis (inflammatory tendinopathy) or tendinosis (degenerative tendinopathy), mainly caused by overuse, resulting in pain and functional limitations that negatively impact the patient’s daily activities. Today, more information is available on the pathophysiological processes of tendons, which allows for more specific treatments that alleviate symptoms and address the underlying problem. Tendinopathy can be treated through various options such as relative rest, physiotherapy, nutritional changes, surgery, regenerative medicine, pharmacology, which is the most widely used, and new techniques that use polymeric materials to treat musculoskeletal pathologies2)(3.
Tendinopathy is a challenging condition to treat. An incorrect diagnosis and indiscriminate use of anti-inflammatories and analgesics can lead to degeneration of the tendon and loss of its mechanical properties, resulting in tendon damage and rupture in severe cases2)(3)(4. Systematic reviews of tendinopathy treatments encompass non-invasive and invasive options, depending on the stage of the condition's progression4)(5. The approach to treatment includes various therapies, such as physiotherapy, platelet-rich plasma infiltrations, prolonged rest, drug use, and, ultimately, surgery6. These treatments, such as physiotherapy, primarily aim to reduce pain, stiffness, and tendon thickness and increase mobility range, elasticity, and muscular strength through exercises, manual therapy, thermotherapy, and electrotherapy7. However, these treatments only reduce the patient's pain, and the degenerative issues associated with tendon pathology are often overlooked.
Pharmacological treatment is one of the most utilized approaches for managing musculoskeletal system pathologies. While it may be effective in other pathologies, it presents a challenge in tendinopathy due to the low blood supply to the tendon. As a result, higher doses are required to achieve therapeutic effects, which can increase the progression of the disease. Moreover, there is a limited understanding of the pathophysiological mechanisms that cause tendinopathy, which adds to the complexity of managing the condition and impeding patient recuperation8.
Hydrogels are promising materials in the pharmacological treatment of tendinopathy, derived from natural or synthetic polymers with a structure that resembles a three-dimensional porous network capable of retaining significant amounts of water9. This retention capacity makes them attractive as support and drug-releasing agents at the injured site. Hydrogels as drug carriers can prevent drug denaturation and aggregation after exposure to inorganic solvents, helping to avoid enzymatic and environmental degradation of the drug10)(11. In addition, they provide a favorable environment for cell or drug infiltration, compensating tendon damage5)(12)(13)(14)(15. On the other hand, studies have shown that hydrogels also maintain tissue homeostasis, promoting faster tissue recovery in in vitro and in vivo models16)(17)(18.
The use of polymer hydrogels is highlighted due to their numerous characteristics and advantages, in contrast to conventional methods, as supports for drug administration in the management of degenerative tendinopathy. Given the necessity for drug delivery strategies in tendinopathy, hydrogels present an attractive alternative with potential application in this biomedical field18)(19. The relevance of hydrogels in tendon pathology lies in the fact that, thanks to their properties, they can simulate the tissue structure, replace damaged tissue without the body rejecting it, and also act as a support to store drugs that stimulate repair at the cellular level of the damaged area, controlling the speed and the desired dose20)(21)(22)(23. This work offers a review of the literature on the pathophysiology of tendinopathy, the challenges involved in its treatment, the utilization of hydrogels, and the advantages they provide as drug carriers.
Materials and methods
A literature review of articles was carried out to collect information on tendon pathology, biopolymers, hydrogels, and drug carriers, using the keywords: tendinopathy, tendon regeneration, tissue engineering, composite, stimuli-responsive polymers, and polymer-drug complex. The databases consulted included: Medline, Web of Science, PubMed, ScienceDirect, and Google Scholar. This review covers articles published during the last 10 years; and reviews the title, abstract, results, and conclusion of each publication according to the following selection criteria: 1. The study must contain information on the current challenges of tendinopathy and their treatments, 2. The work must report on hydrogels as treatments for tendinopathies, 3. The research must include the applications of hydrogels for administering drugs to the tendon. In accordance with the above, 200 articles were reviewed, and 90 were selected, of which 20 met the selection criteria, and 70 served to support the related fundamental concepts.
Results and discussion
Tendinopathy
Tendons are part of the musculoskeletal system and transmit force from the muscle to the bone, facilitating joint movement [13]. Tendons are composed of collagen (30 %), extracellular matrix (68 %), and elastin (2 %), which give them their mechanical properties24. Tendinopathy is a degenerative pathology in the tendon, regularly associated with age but also occurring in young people. It is a tendon injury mainly caused by overuse, leading to microtrauma and degeneration3)(6.
The prevalence of tendinopathy has increased worldwide since the early 2000s5, resulting in long-term permanent functional deficits in athletes and sedentary individuals of all ages. Furthermore, its incidence varies between different anatomical regions, with joints with greater mobility being more commonly affected. Several factors, such as age, sex, type of sport, and environment, can influence a patient's predisposition and recovery4. Chronic tendon damage is characterized by a disorder of collagen fibers, as well as the presence of type III collagen fibers13 and increased expression of metalloproteases (MMP) MMP-1, MMP-3, MMP -9 (collagenases, gelatinases, and stromelysins) responsible for the degradation of the extracellular matrix and the limited expression of MMP inhibitors25. While tendons continuously undergo degradation and regeneration to maintain homeostasis and attempt self-repair in the event of damage, the degenerative process progresses if the underlying causes persist26. MMPs are zinc hydrolases involved in physiological functions such as organogenesis, healing, uterine involution, as well as pathological conditions like inflammatory processes, autoimmune diseases, and carcinogenesis. Various cell types, including polymorphonuclear leukocytes, keratinocytes, monocytes, fibro-blasts, and mesenchymal cells, generate MMPs in the presence of growth factors. MMPs are secreted as inactive proenzymes into the extracellular milieu and require activation in the pericellular environment of tissues27. In degenerative tendinopathy, hyperactivity of MMP-1, MMP-8, and MMP-13 degrades extracellular matrix elements such as tendon collagen. The body has endogenous inhibitors of MMPs in tissue (TIMPs) to help repair degeneration and maintain tendon homeostasis, but often they are insufficient to control MMP activity28.
Treatments for tendinopathy
Pharmacological treatments utilize different routes of administration, including intravenous, intradermal, nasal, and oral. The latter is the most used route, but it may not always be the most appropriate for certain pathologies or patients due to the associated disadvantages, among which an incorrect biodistribution stands out. This can require higher doses to achieve the desired effect, especially in poorly irrigated such as tendons10)(29. One major obstacle faced by drugs intended for tendinopathy is poor vascularization, which restricts the drug’s access through the blood-stream and can cause toxicity when high doses are required to achieve the desired effect30. Pain and inflammation also contribute to movement restriction, making recovery more challenging.
The most used drugs for tendinopathy administered orally or intramuscularly are non-steroidal anti-inflammatory drugs (NSAIDs) and analgesics. NSAIDs inhibit cyclooxygenase activity, decrease the synthesis of proinflammatory prostaglandins involved in the inflammatory response, and increase tissue permeability and blood flow. However, prolonged use can lead to side effects such as gastrointestinal, renal, and cardiovascular disturbances31. Corticosteroids are less frequently used for tendinopathy but have shown beneficial effects in the short and medium term, such as in infiltrations for chronic tendinopathy of the middle portion of the achilles tendon.
Tissue engineering represents an alternative approach used to investigate tissue injury repair. Biomaterial-based drug carriers have been proposed, with natural polymers being the preferred choice due to their bio-compatibility, high availability, and low cost. Similarly, synthetic polymers like polylactic acid have been suggested for their adaptability, reproducibility, and low immunogenicity32. These biomaterials should possess adequate architecture, good biomechanical performance, and be bioresorbable, biomimetic, biodegradable, biocompatible, and exhibit low immunogenicity. Natural polymers, such as collagen, gelatin, and cellulose, have been evaluated for tendon regeneration due to their availability and affordability, and they have shown promising results in both in vitro and in vivo studies33.
Hydrogels and their relationship with the tendon extracellular matrix
The mechanical characteristics of the extracellular matrix have been evaluated in terms of elasticity, which is the resistance of an object to undergo reversible deformation in response to an external force. Thanks to its high-water content, the extracellular matrix is viscoelastic, and viscoelasticity plays a fundamental role in matrix mechanics34. Due to matrix composition and organization changes, the response to mechanical stress and strain differs between human body organs35. Viscosity is a property that arises from a fluid's resistance to deformation. The combination of elasticity and viscosity results in time-dependent stress dissipation, known as viscoelasticity36. Unlike materials that possess only elasticity and store and retain energy, viscoelastic materials can dissipate energy in the face of time-related stresses, making the elastic modulus dependent on the strain rate. Thus, viscoelasticity is an intrinsic property of the extracellular matrix recognized in biological systems37. As previously mentioned, tendons tend to break due to the tissue composition and the constant load they experience, making it essential to pay attention to their mechanical properties. Deepthi, et al.38 used a layered chitosan-collagen hydrogel to mimic native extracellular matrix glycosaminoglycans for tendon regeneration. These results showed that layered chitosan-collagen hydrogel is ideal for tendon regeneration and for preventing tendon adhesion.
Hydrogels are water-swollen polymeric networks (Figure 1) that are used as in vitro systems to simulate the extracellular matrix9. When producing hydrogels, it is necessary to consider the raw materials, which are mainly natural or synthetic polymers. Additionally, the appropriate crosslinking technique, whether physical or chemical, must be chosen. Physical crosslinking does not require a crosslinking agent for the reaction to occur since the hydrogel is in a liquid state, and a change in the environment, ionic concentration, temperature, or pH trigger the gelation. On the other hand, chemical crosslinking occurs through a crosslinking agent, enzymatic reactions, radiation, click reactions, or polymerization, and these processes are irreversible39)(40.
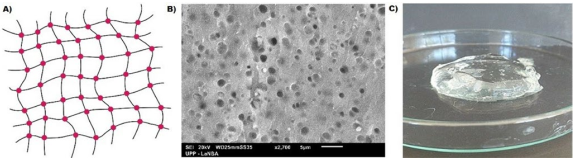
Figure 1 A) Representation of the macroscopic structure of the hydrogel. B) Surface morphology of a hydrogel observed by Scanning Electron Microscopy C) Carboxymethylcellulose hydrogel.
Regulating the mechanical characteristics of hydro-gels is essential for assessing their suitability in tissue applications. Mechanical properties, such as viscoelasticity, depend heavily on the polymer structure, type, molecular weight, swelling index, and crosslink density.
The hydrogels must have adjustable viscoelastic properties since the similarity with the extracellular matrix of the tissue depends on this, allowing cellular regulation and diffusion of drugs, depending on the method used for the application. Therefore, the hydrogels must have adequate stress relaxation kinetics that matches what occurs in biological processes in vivo41. Sun, et al.42 report tendon-mimetic hydrogels constructed from anisotropic assembly of aramid nanofiber composites and had a high modulus of ~1.1 GPa, strength of ~72 MPa, fracture toughness of 7333 J/m2, characteristics matching those of natural tendons.
With the rise of biomaterials, viscoelastic hydrogels have been designed to construct a dynamic mechanical microenvironment that more closely mimics the mechanics of the native extracellular matrix. Native tissues behave as viscoelastic materials and play critical roles in maintaining physiological activity, such as energy dissipation by tendons during daily movement and exercise, protecting them from continuous or periodic high stresses or strains41. Tissue bioengineering has developed collagen I hydrogels, and it has been emphasized that the mechanical characteristics of hydrogels must match those of tissues to achieve adequate physiological behavior43. The mechanical characterization of viscoelasticity can be carried out through dynamic mechanical analysis in tension, compression, and torsion. However, such data for living tissues are sometimes unavailable. For instance, tendons and muscles undergo rapid stresses and relatively high strains and are anisotropic, whereas most tissues undergo relatively small stresses under physiological conditions and exhibit linear behavior.
Hydrogels continue to be studied because their mechanical properties can be improved for specific applications. Chou et al.44 prepared thermosensitive hydrogels-based poly(N-isopropylacrylamide) in situ to prevent postoperative peritendinous adhesion. This provides mobility and flexibility during injection application, allowing for gap-filling after surgery. Nevertheless, synthetic hydrogels have low gel strength, poor tenacity, and slow water absorption.
It is important to mention the swelling capacity of hydrogels, especially in cases where the drug is released through swelling. In these instances, the drug is dispersed within a polymer, and when it comes into contact with a fluid, the polymer starts to swell45. The swelling rate is calculated by measuring the change in weight before and after water absorption, expressed as a percentage of weight gain. In swelling-controlled delivery systems, the higher the rate of hydrogel swelling, the higher rate of hydrogel swelling corresponds to a higher rate of drug release. For example, a novel injectable doxorubicin-loaded hydrogel achieved equilibrium swelling within 72 h with high swelling ratios ranging from 3,500 % to 4,000 %, depending on the pH values of PBS solutions46.
Porosity is another crucial characteristic as it simulates tissue permeability for cell growth or migration or as a support. This characteristic is defined by pores of different sizes, shapes, spatial distribution, and interconnections47. Hydrogels exhibit limited internal diffusion, wherein a hydrogel placed in a liquid medium swells or collapses until it reaches equilibrium due to the balance between the osmotic forces originating from water entering the macromolecular network and the cohesive-elastic forces exerted by the polymeric chains that oppose this expansion48. Likewise, the porosity of the hydrogel is an essential physiological factor as it promotes the transport of oxygen and nutrients to maintain cell survival. Additionally, it acts as a reservoir with a high loading capacity for therapeutic actives (drugs, proteins, among others), protecting them from environmental degradation and subsequently releasing them through various mechanisms14.
In such a way that the pore is an important parameter that can affect drug diffusion. Optimal porosity is necessary for increased drug release, which is supposed to depend on the size relation of hydrogel pores. However, it has been observed that very high porosity does not necessarily result in increased drug release. Pore size distributions narrow as the concentration of the polymer chosen for the hydrogel design increases. Such is the case of a polyethylene glycol methacrylate hydrogel. Pore sizes were determined from bulk hydrogels by cryo-SEM. Smaller pore sizes (30-150 nm) were obtained by increasing polymer concentration due to an increase in network density. They concluded that small substances encapsulated in hydrogels prepared with low concentrations of polymer show an initial burst release. They observed a very slow initial and accelerated release starting on day 6. This indicates that a fraction of the substance resides in the large pores from which diffusion could rapidly release it49.
The size of hydrogel pores can be modulated by controlling the crosslink density50. These interconnected pores vary in size from a few micrometers to hundreds. Analyzing hydrogel pores presents a challenge in accurately determining their size and distribution47. It is necessary to consider that the pore sizes are more significant for proper storage and subsequent release of any loaded substances, such as drugs or biomolecules51. Similarly, the morphology of the hydrogel affects the degree of degradation. In porous hydrogels where the pore size exceeds the molecular dimensions of the drug, the diffusion coefficient is related to the hydrogel´s porosity and tortuosity52. In hydrogels with pore sizes similar to the molecule size or non-porous hydrogels, the drug diffusion coefficient decreases due to steric hindrance caused by the polymer chains. In such cases, the volume available for mobility decreases, and the hydrodynamic resistance increases, resulting in an increased drug diffusion path length compared to hydrogels with larger pore sizes53.
According to Stoppato et al.21, injection of a 2-meth-acryloyloxyethyl phosphorylcholine hydrogel around the tendon in a murine model with achilles tendinopathy and a chicken model with deep flexor digitorum tendinopathy resulted in a hydrogel with nanometer-sized pores. When injected, it would allow the penetration of signaling molecules, inhibiting tendon filtration and cell adhesion without adverse effects.
Finally, regarding the hydrogel's physical and chemical degradation, physical degradation provides a space for cell migration and vascular infiltration for tissue regeneration54. The degree of hydrogel degradation must match the degree of new tissue formation to maintain tissue integrity and mechanical properties. The physical degradability of hydrogels depends on the properties of the materials that compose them and the microenvironment conditions23. To ensure tissue regeneration, the degradation process must be synchronized with cell proliferation and blood vessel infiltration55. After being placed, hydrogels begin to degrade, directly impacting the controlled drug release and the final result of tissue repair. It is essential to control the degree of degradation of hydrogels, which is determined by the crosslinking density of the network that forms the hydrogel56. Silva et al.57 report a photocrosslinkable magnetic-responsive hydrogel composed of methacrylated chondroitin sulfate and enriched with platelet lysate for application in the ten-don-to-bone interface; hydrogel degradation was completed in 17 days. Sundaram et al.58 developed a chitosan hydrogel reinforced with twisted poly (L lactic acid) aligned microfibrous bundle to mimic the tendon extracellular matrix. The degradation pattern was observed over time due to the degradation of the chitosan hydrogel layer from the final construct. Chitosan is a polymer that has been extensively studied, and its degradation rate can range from six months to a year. The degradation occurs via hydrolysis or enzyme attack.
Therefore, it is important to design hydrogels that mimic the properties of the extracellular matrix and the changes to which it will be subjected. Additionally, it is necessary to study these hydrogels according to their characteristics to replicate them in an in vivo model of tendon pathology.
Hydrogels as carriers for drug delivery
Routes of drug administration, such as nasal, intravenous, intradermal, and oral, are utilized, with the latter being the most common. However, due to various obstacles, oral administration may not always be the best option for all patients or diseases. These obstacles include low biodistribution, especially in poorly irrigated tissues such as tendons, where the drug will take a long time to reach the target site, low solubility, high toxicity at larger doses, and short circulation times in the blood (less than 12 hours)10. Conventional pharmaceutical formulations include tablets, capsules, injections, patches, sprays, and drops. Nevertheless, in tendinopathy, minimal irrigation makes it difficult to control plasma concentration and renders these treatments inefficient59.
Hydrogel-based drug delivery systems overcome the barriers of conventional methods and contribute to effective patient treatment. These systems enable the control of drug availability in tissues for extended periods. They have been developed to modify administration routes to benefit patients, improve bioavailability, and alter release profiles60. Dong et al.61 reported the development of an injectable cyclodextrin-adamantane hydrogel to encapsulate and modulate insulin release in vivo and in vitro for 30 days.
Furthermore, hydrogels are an alternative in drug delivery to injured areas due to their soft, elastic consistency, high water content, and important interaction with living tissues. Some hydrogels are inert materials, so cells and proteins do not adhere to the surface59. In this context, Prucker et al.62 developed a poly-dimethyl-acrylamide hydrogel with a blood protein-repelling surface. However, some hydrogels can adsorb proteins and cells and may remain on the surface or migrate depending on the application16. Cell adhesion into the hydrogels can be controlled via selection of carbohydrates with defined physical properties (hydrophilicity, charge) and biological properties63. For example, a chiral hydrogel accelerates re-epithelialization in chronic wounds and facilitates keratinocyte adhesion, proliferation, and migration, which accelerates re-epithelialization64.
The swelling behavior of hydrogels allows them to absorb, retain, and release active substances under controlled conditions. The high water content makes them compatible with most living tissues45. Mater et al.65 mentioned in their patent the development of a polyethylene glycol hydrogel with adjustable swelling indices to release silver ions as an antimicrobial agent in biomedical applications.
Hydrogels, as a means of drug delivery, should allow for continuous drug administration at the necessary concentration level, achieving optimal performance during therapeutic action. The concentration must be less than the toxic level and greater than the minimum efficiency to be considered optimal. The main problem with conventional administration systems is the harmfulness of treatments due to the use of higher doses to attain the desired outcomes. The challenge for existing therapies is to achieve optimal concentration control in a single dose of a more significant volume, thereby eliminating the need for daily doses. In this sense, hydrogels are promising treatments5.
Hydrogels offer numerous benefits as drug delivery and release agents compared to traditional techniques. Short-term drug administration frequencies can lead to better patient reactions to the treatment, which is more practical. In addition, there is a decrease in the fluctuation of plasma concentrations, especially with rapidly absorbed drugs, which causes a reduction in high plasma peaks, minimizing adverse effects and, in turn, avoiding subtherapeutic plasma levels that lead to the loss of efficacy66. Additionally, hydrogels can protect drugs against environmental and enzymatic degradation and can transition from a liquid state to a gel state for in vivo injection, resulting in improved drug utilization and a reduced need for frequent dosing67.
Typically, the active substance is chemically attached to the hydrogel in these drug delivery systems. However, the most common method is to swell the hydrogel with the drug solution to prevent denaturation of the drug and physically bond it to the hydrogel. The hydrophilic properties of the hydrogel trap the drug in solution form, acting as a storage and dosage support11. For example, Malik et al.68 developed a carboxymethylcellulose hydrogel cross-linked with β-cyclodextrin to control the release of acyclovir. The hydrogels were dried and immersed in the acyclovir solution to add the drug, then frozen for subsequent freeze-drying.
In some cases, the drug is chemically attached to the polymer chains and subsequently released through hydrolytic or enzymatic degradation11. The degradation rate and gelation of the hydrogel are important factors to consider for therapeutic applications, particularly in tissue regeneration. For instance, when a hydrogel is used for tendon regeneration, its gelation and degradation rates can impact its mechanical and biological properties and must align with tissue remodeling69. Kuo et al.70 developed a xanthan gum/gellan gum/hydrogel to reduce tendon adhesion without compromising tendon strength. The material’s degradation rate increased, and the membrane acted as a barrier for an extended period of time. Due to their characteristics and design, hydrogels have been shown to overcome the limitations of conventional drug administration routes and offer advantages in treating tendinopathy. Therefore, hydrogels represent a promising treatment with a lower probability of producing adverse effects compared to conventional routes.
Mechanisms of drug release in hydrogels
Hydrogels can release drugs through diffusion, swelling, or chemical erosion (Figure 2). From a biomaterial perspective, the goal is to develop systems loaded with the active ingredient/substance and respond by releasing their load at the desired place, time, and rate. For this reason, hydrogels have gained popularity as they can be tailored to meet the specific needs of patients and pathologies18. Hsiao et al.71 developed a hyaluronic acid hydrogel as a drug carrier for early inter-vention in tendinopathy. The hydrogel demonstrated an initial drug release burst of 51.5 % during the first 24 h, followed by a steady release of 40.8 % over 1-10 days.

Figure 2 Mechanisms of hydrogel drug release. a) Chemically by homogeneous erosion, b) chemically by heterogeneous erosion, c) Dissemination from a matrix system, d) dissemination from a reservoir system, e) Diffusion by swelling.
Release by diffusion: This is the most common mechanism, which allows molecules to move from a higher solute concentration zone to a lower concentration zone when separated by a polymeric membrane. It is essential to consider the size of the hydrogel pores. When the pores are larger than the drug, the speed of dissemination is related to the hydrogel's porosity. However, when the pore size is similar to the drug, the speed decreases, resulting in reduced volume available for mobility and increased path length for drug dissemination72. Freedman et al.73 report adhesive hydrogels that facilitate the diffusion of a drug from the hydrogel drug reservoir into the target tissue rather than in the adjacent tissues by increasing the direct contact surface area and reducing the diffusion barrier.
Release by swelling: The influx of solvent molecules that swell the hydrogel controls the release. In these systems, the drug is dissolved or dispersed in the polymers in either a crystalline or vitreous state. There is a transition from the crystalline form, where the molecules are immobile, to a gel-like state, where the molecules can quickly disseminate11. For example, Pourjavadi and Doroudian74 synthesized an electro-responsive hydrogel containing biodegradable and hydrolyzed collagen polypeptide. The drug release was achieved through hydrogel swelling.
Release by chemical degradation: Chemical erosion can be classified into homogeneous and heterogeneous erosion, determined by the polymer’s morphology and hydrophobicity. Homogeneous erosion occurs through-out the hydrogel, while heterogeneous erosion occurs only on the surface. The more hydrophilic the polymer, the more homogeneous the erosion, as it can absorb more water. Conversely, the more crystalline the polymer, the more heterogeneous the erosion, as the crystalline regions exclude water. Drug release is generated through enzymatic degradation or hydrogel swelling, exposing the drug to the surrounding medium. In systems with lateral chains, the drug is chemically bound to the polymer chains and later released through hydrolytic or enzymatic breaking of the linkages11. Zheng et al.75 developed sequentially degradable injectable hydrogel microspheres for synergistic local chemotherapy. Combretastatin A-4 was released from the hydrogel with more rapid degradation, perturbing the vascular structure of the tumor and reducing the exchange between the tumor and adjacent tissues.
As has been observed, hydrogels can be tailored to deliver drugs based on the specific needs of tendon pathologies. Therefore, their characteristics and design can be modified to ensure proper drug dosing and distribution within the damaged tissue.
Hydrogels used in tendinopathy
In the pathology of tendons, no treatment has obtained long-term results, and the tendons do not regain their original strength and function. Thus, it is essential to develop materials that mimic tissue characteristics, transport cells, growth factors, and drugs to the damaged area, and aid in tissue regeneration without causing inflammatory reactions or adhesions19)(76)(77. In addition, these materials must be stable and possess good mechanical properties, biocompatibility, and bio-degradability78. Farnebo et al.79 developed a tendon-derived extracellular matrix hydrogel to enhance tendon healing in vivo. The hydrogel was infused with fibroblast growth factor, insulin-like growth factor-1, and platelet-derived growth factor-BB, which stimulated cell proliferation.
Hydrogels used in tendinopathy
In the pathology of tendons, no treatment has obtained long-term results, and the tendons do not regain their original strength and function. Thus, it is essential to develop materials that mimic tissue characteristics, transport cells, growth factors, and drugs to the damaged area, and aid in tissue regeneration without causing inflammatory reactions or adhesions19)(76)(77. In addition, these materials must be stable and possess good mechanical properties, biocompatibility, and biodegradability78. Farnebo et al.79 developed a ten-don-derived extracellular matrix hydrogel to enhance tendon healing in vivo. The hydrogel was infused with fibroblast growth factor, insulin-like growth factor-1, and platelet-derived growth factor-BB, which stimulated cell proliferation.
Hydrogels must be biocompatible materials due to potential immunological reactions in the body. These materials have the ability to mimic the composition and structure of the tissue's extracellular matrix, thereby reducing the immune responses and the risk of rejection by the patient20.
Hydrogels serve as an excellent tool for controlling regeneration, acting as cellular support for the development of engineered tissue in vitro or as a delivery vehicle for in vivo therapeutic agents, such as cells or bio-molecules. Hydrogels provide temporary scaffolds composed of the tendon's extracellular matrix. For instance, tendons can be engineered using hydrogels loaded with cultured cells in bioreactors under mechanical conditions to stimulate tenogenesis and generate tissues in vitro prior to implantation21. Another example involves the use of hydrogel microspheres to deliver drugs, cells, or bioactive factors, with the encapsulated contents exhibiting long-acting and sustained release effects that extend degradation time11. In another report, composite scaffolds formed by combining hydrogels with other materials facilitated viable cells and growth factors with improved mechanical properties, such as collagen gels combined with poly(3-hy-droxybutyrate-co-3-hydroxyhexanoate) tubes. The tis-sue compatibility of the polyester and its delayed biodegradability promoted cell migration, organization, and function in a murine model of achilles tendon repair80.
Delivery vehicles: Hydrogels that deliver therapeutic agents into the tendon can enhance tissue healing or regeneration. An example of this is a sustained-release hydrogel-based Rhynchophylline delivery system used to repair injured tendons22. Xu et al.81 developed an injectable fibromodulin-releasing hydrogel for tendon healing that significantly improved the histological results of tendon wound healing and led to the recovery of mechanical properties. Various cell delivery methods, such as hydrogel-based cell delivery, which delivers dermal fibroblasts into the tendon, can enhance and stimulate this process compared to adipose-derived stem cells82.
Injectables: The production of injectable hydrogels for repairing and regenerating tendons uses natural and synthetic biomaterials since it is necessary to consider biological parameters, such as cell adhesion and material degradation, as well as physical parameters, like mechanical properties. Due to their advantages over synthetic materials, the most commonly used natural materials include collagen, fibrin, gelatin, chitosan, and hyaluronic acid. However, these natural materials degrade quickly, and their mechanical properties are insufficient. Injectable hydrogels have gained relevance in numerous applications. Injuries to the musculoskeletal system, especially tendons, present a significant challenge as they do not respond adequately to conventional treatments, and damaged tissue is slow to repair, rarely achieving full recovery80. In situ injection can treat tissue with minimal damage and side effects15. Most hydrogels can be injected in situ into custom-shaped molds or scaffolds to form composite structures (Figure 3)21.
A patent has been proposed for an extracellular matrix hydrogel obtained from mammalian connective tissue to facilitate the infiltration of fibroblasts, tenoblasts, and tenocytes and promote the repair and regeneration of tendons83. Likewise, a biocompatible hydrogel comprised of a human tendon extracellular matrix (ECM) allowed the creation of a tendon graft through the photocrosslinking of stacked scaffold sheets84. In this regard, cellulose hydrogels have been increasingly studied for tissue regeneration due to their similarity to ECM85.
However, the progress of injectable hydrogels, whether derived from natural or synthetic polymers, for tendon repair highlights the need to develop hydrogels with better mechanical properties, specific binding to tissue damage, and disease response to achieve personalized treatment86. Current treatments using hydrogels as supports or delivery vehicles are suitable for use in tendinopathies, despite their limitations21. For instance, Kim et al.87 reported on a hyaluronic acid-based injectable hydrogel for tendinopathy treatment, using vitamin D on damaged tenocytes as a new regeneration technique and demonstrating its capability to repair tendinopathy based on tendon restoration properties in vitro and in vivo. However, a more advanced delivery system could be developed with sophisticated scaffolds such as hydrogels and nanofibers.
The main purpose of using hydrogels in tendinopathy is to deliver the drug to the specific site of the lesion due to the limited capacity of the tissue for self-repair.
Hydrogels function as supports and delivery systems for the active agents in the damaged area. Overall, the progress made in the use of hydrogels for tendon repair highlights the potential for developing more advanced delivery systems in the future.
To quantify the effectiveness of hydrogels as a tendinopathy treatment, the Bonar Score has been designed to assess tendon morphology and histological changes such as cell morphology, collagen arrangement, cellularity, vascularity, and ground substance88. Alternatively, the Victorian Institute of Sport Assessment (VISA) scale can be used to analyze the results in the patient's clinic. Alternatively, the Victorian Institute of Sport Assessment (VISA) scale can be used to analyze the results in the patient's clinic. It is useful for assessing pain, stiffness, body structure or function, activity limitation, and restriction of the patient's participation in daily living activities or sports6.
Finally, Table 1 summarizes the data corresponding to the hydrogels reported in this document that have been directly applied to tendon pathologies.
Table 1 Advances in the treatment of tendons with hydrogels
System | Applications | Properties/results | Ref. |
---|---|---|---|
ADN Hydrogel | Delivery system for tendon stem/progenitor cells | Hydrogel promotes cell proliferation and protection | 12 |
3D printed hydrogelparticles containing PRP | Tendon postinjury repair | Hydrogel promotes tendon differentiation in tendon-derived stem cell and reduces inflammatory response | 16 |
Gelatin/Fe3O4/Celecoxib hydrogel | Repair the later stage in tendinopathy | Hydrogel reduced inflammatory reaction of macrophage response | 19 |
Chitosan-collagen HG with poly-L-lactide fibers | To mimic the glycosaminoglycans of sheath extracellular matrix for tendon regeneration | Good cell proliferation was observed, tenocytes showed proper attachment and spreading on the scaffolds, indicating that they would be suitable for flexor tendon regeneration | 38 |
Collagen I hydrogels | Bioengineered Tissue microenvironments | Scaffolds for engineered tissues with a diffusion capacity | 43 |
Thermo-responsive in-situ HG based on poly (Nisopropylacrylamide) | Prevent post-operativeperitendinous adhesion | Hydrogels were feasible as injectable barrier materials to prevent postoperative peritendinous adhesion and maintain the intra-tendinous strength or repaired tendons | 44 |
Synthetic Polymer, Natural polymer-based hydrogels | Wound healing, adsorbents, drug delivery | CMCN can absorb large amounts of water and form superabsorbent hydrogels depending on the crosslinking agent used | 48 |
Photocrosslinkable magnetic responsive HG made of methacrylate chondroitin sulfate with platelet lysate | Application in tendon-tobone interface | In addition to swelling and electromagnetic fields, degradation could modulate the release profile of growth factors, and therefore could be used as a delivery strategy | 57 |
Xanthan gum/gellan gum/hyaluronan HG membranes | Prevent the adhesion of postrepaired tendons | The HG membranes degraded slowly, reducing tendon adhesion without compromising tendon strength | 70 |
Drug-loaded hyaluronic acid hydrogels | Sustained release in early intervention of tendinopathy | Better recovery of the tissues and faster healing promoting oxidative stress migration | 71 |
A tough adhesive HG, termed Janus Tough Adhesive | Drug delivery for tendon | HG adheres to tendons, is biocompatible, promotes tendon healing, and enables high drug loading and sustained drug release in silico and in vitro | 73 |
Polyvinyl-alcohol and hyaluronic acid hydrogel for controlled drug release | Promote tendon healing | Hydrogel alleviated inflammationenhancing tendon wound regeneration | 76 |
Sustained-Release hyaluronic acid hydrogelbased delivery system | Prevent adhesions astreatment for injured tendons | Local release inhibits the formation of tendon adhesion and help the injured tendon to structural healing | 77 |
2-methacryloyloxyethyl phosphorylcholine HG | Prevent the formation of adhesions in the tendon | The HG prevented tissue adhesion to the tendon with no adverse effects on the healing process of the injured tendon | 78 |
Tendon-derived extracellular matrix HG | Tendon healing in vivo | The extracellular matrix hydrogel with growth factors stimulates cell proliferation, and infiltration of host cells into the gel increases with an optimal concentration of growth factors in vivo | 79 |
Injectable granular hyaluronic acid HG | Tendon healing and recovery of functions | The tendon strength of the treated rats and their gait performance returned to normal, similar to the healthy controls | 81 |
HG- cadaver tendon | Delivers dermal fibroblasts into the tendon | It enhances and stimulates this process compared to adipose-derived stem cells | 82 |
Decellularized extracellular matrix HG derived from mammalian connective tissue | In-situ repair and regeneration of an injured ligament or tendon in mammalian subject | It facilitates fibroblast, tenoblast, and tenocyte infiltration and promote ligament or tendon repair and regeneration | 83 |
A biocompatible HG consisting of extracellular matrix (ECM) from human tendons | Tendon tissue engineering | Decellularized tendons were milled and enzymatically digested to form an ECM solution, which offers a promising alternative in tendinopathies | 84 |
Injectable hyaluronic acidbased HG | Restoration of tendinopathy | The hydrogel facilitated anti-apoptosis, tenocyte proliferation, tendon-related protein production, and tissue alignment during the regenerating tendon process | 87 |
Conclusions
The approach to tendinopathy through multidisciplinary health sciences and technologies has made it possible to break down the barriers of conventional treatments, ranging from physiotherapy, drugs, and surgery to tissue limitations. The high incidence and poor management of tendinopathy have generated greater interest and research. In this sense, natural or synthetic polymer hydrogels are promising smart materials that offer multiple advantages. Their improved mechanical properties allow the restoration of injured tendon tissue and also act as supports and controlled drug-releasing systems in low-irrigated tis-sues such as tendons, accelerating their recovery. Hydrogels, as drug carriers, hold promise for treating tendinopathy and continue to be incorporated into new treatment strategies. For future applications, it is necessary to evaluate hydrogel release systems in a clinical environment and assess their long-term effectiveness in vivo.
Future directions
The future directions of hydrogels are based on the modulation of macrophages and immune cells, controlling their reprogramming and plasticity to regenerate tissues. Additionally, designing intelligent regenerative hydrogels as tissue engineering tools to guide the growth, differentiation of cells, and spatial organization20. Developing hydrogels with mechanical properties specific to tissue damage and responsive to the disease is necessary. In the same vein, the advantages of various biological materials should be combined to achieve personalized therapy59. Furthermore, it is necessary that hydrogels as drug delivery systems be further studied to improve their sustained release properties, ensuring the correct doses of the drug are stored to maintain an optimal drug concentration over time. Finally, the application frequency on the human body should be optimized89)(25.