Introduction
Large quantities of catalysts are used in the petroleum refining industry to produce clean fuels and many other valuable products (Marafi, Stanislaus & Furimsky, 2010; Stanislaus, Marafi & Rana, 2010), being the spent hydro-processing catalysts the major solid waste generated by refinery industries (Liu, Yu & Zhao, 2004). The production of this kind of residues has significantly increased in recent years due to the rising need of heavy oil processing, together with the accelerated demand of low Sulphur fuels (Petróleos Mexicanos (PEMEX), 2002; Shen, Shen & Ling, 2013). The catalysts which consist of Mo with promoter Co (Ni) on alumina support enhance the removal of undesirable impurities by hydrodesulfurization, hydrodenitrogenation, and hydrodemetallization reactions, respectively. Hence, catalysts used for this process are deactivated rapidly by coke and metal (V and Ni) deposits and have a short life (6 months - 12 months) (Al-Sheeha, Marafi, Raghvan & Rana, 2013). Between 150 000 t - 170 000 t of spent hydroprocessing catalysts are generated world-wide annually (Dufresne, 2007; Marafi & Stanislaus, 2008), which contains diverse metallic hazardous components such as Al, V, Mo, Co, Ni, As and Fe, as well as nonmetallic elements like elemental sulfur, carbon and oil (Marafi & Stanislaus, 2007; 2008). Due to their risky nature in health and environmental issues, the handling of spent catalysts in Mexico is regulated according to the Mexican Standard established in NOM-052-SEMARNAT-2005 (Diario Oficial de la Federación [DOF], 2006). As settled by the Environmental Protection Agency (USA), the Organization for Economic Cooperation and Development (OECD), and the Basilea Agreement, each country is responsible for the generation, safe disposal or recycling of these materials (Marafi & Stanislaus, 2008). Hence, it is important to develop viable alternatives for the safe reutilization, treatment or disposal of spent catalysts.
Both hydrometallurgical and pyrometallurgical methods are available for metal recovery from spent catalysts (reviewed by Akcil, Vegliò, Ferella, Okudan & Tuncuk, 2015). However, these processes generate large volumes of potentially hazardous wastes and emission of harmful gases (Llanos, Lacave & Deering, 1986). In contrast, biological methods, which are considered as viable environmental-friendly technologies, have been successfully developed in the last years and have been associated with lower cost and energy requirements, in comparison to non-biological processes (Oza & Patel, 2012).
Microorganisms can detoxify metallic components by two different strategies: 1) by an active process of metal removal conducted by living cells, which generally involves mechanisms of bioleaching, precipitation or sequestration (Gupta & Keegan, 1998); or 2) by passive means, involving the metal biosorption on living or dead biomass (Churchill, Walters & Churchill, 1995).
In the other hand, reports have shown that growth environmental modifications such as temperature, pH and aeration can affect adaptation responses and survival of Bacillus species (Budde, Steil, Scharf, Völker & Bremer, 2006; Foda, Salama & Selim, 1985; Raevouri & Genigeorgis, 1975). As well, the presence of diverse carbon sources can cause drastic changes in the structure of gram positive microorganisms, by altering the ratio of the cell wall components (Tipper & Wright, 1979), consisting mainly of polysaccharides, proteins, lipids, and peptidoglycan; which may interact with the metals present in the environment (Ozturk, 2007). Therefore, the basis for these differences as well as for variations in metal-accumulation abilities may be the result of cell surface characteristics (Edyvean, Williams, Wilson & Aderhold, 1997; Tsezos, 1997). The alteration of the metal uptake abilities of some microorganisms by the presence of a carbon source has been previously demonstrated (Hassan & El-Kassas, 2012). For example, it was observed that the addition of carbon sources resulted in a higher binding capacity of the metal ions Fe(III) and Cr(VI) by S. cerevisiae (Goyal, Jain & Benerjee, 2001). In contrast, the addition of succinate (0.5% w/v) reduced nickel uptake by Pseudomonas aeruginosa by 0.5-fold (Sar, Kazy, Asthana & Singht, 1998). Additionally, carbon sources may be considered as metal chelators (Bourne, Searle & Weigel, 1971), and the sequestration of cupric, calcium, strontium, and ferric ions by glucose and its derivatives has been previously documented (Hodge, Nelson & Moy, 1963).
Thus, based on previous reports and with the aim of studying the effect of PHGII medium and PHGII medium without glucose in the metal removal ability of Bacillus species, the current study reports on the ability of three Bacillus spp. strains to remove Ni and V from a spent catalyst when using PHGII medium or PHGII without glucose, revealing its potential use for the treatment of high-metal-content industrial wastes.
Materials and methods
Microorganisms, culture media and growth conditions
Three different strains identified as Bacillus spp. MV-AH-1 (Gómez-Ramírez, Montero-Álvarez, Tobón-Avilés & Rojas-Avelizapa, 2012), Bacillus megaterium MNSHI9K-1 (Arenas-Isaac et al., 2016), and Bacillus megaterium MV-9K-2 (Gómez-Ramírez, García-Martínez, Fierros-Romero & Rojas-Avelizapa, 2014) were previously isolated from different high metal content sites in Guanajuato, Mexico (Gómez-Ramírez et al., 2012). The analyses were performed at 30 °C and 150 rpm in PHGII medium (casein peptone, 4 g/L; glucose, 2 g/L; yeast extract, 1 g/L); pH was adjusted to 7.0. PHGII solid medium was prepared by adding bacteriological agar (Bioxon, Mexico City, Mexico) at a concentration of 15 g/L (Gómez-Ramírez, Montero-Álvarez, Tobón-Avilés, Fierros-Romero & Rojas-Avelizapa, 2015).
Spent catalyst
The Mexican Petroleum Institute (IMP, for its acronym in Spanish) provided the spent catalyst, named H-oil, which is composed of cylindrical particles of 3 mm - 4 mm long and 1 mm in diameter. Metal composition of H-oil (in mg of component / kg of catalyst) was previously reported as: Al 109713.3 ± 3040.9; V 57617.7 ± 1156.4; Mo 32688.6 ± 892.7; Ni 24822.3 ± 550.1; Mg 316.1 ± 18.3; Fe 279.9 ± 21.2; Zn 199.5 ± 0.2; P 92.0 ± 3.0; As 42.3 ± 0.0; Cr 40.7 ± 0.0; and Cd 0.3 ± 0.10 and 36% (w/w) of oil hydrocarbon (Gómez-Ramírez et al., 2014). For experimentation, the catalyst was pulverized using mortar and pestle and was stored until experimental use. The size particle of spent catalyst was analyzed by using a ZEISS Microscope Axio Scope A.1, and the software AxioVision Rel. 4.8. Catalyst particle size was 945.7 μm ± 264.3 μm long and 734 μm ± 193 μm wide. No treatment was done for the removal of oil hydrocarbons.
Ni and V removal from spent catalyst
Each strain was grown during 24 h to 48 h in 125 mL Erlenmeyer flasks containing 20 mL of PHGII liquid media at 150 rpm and at a temperature of 30 °C. Once the microbial density of each isolate was adjusted to 3 × 108 CFU/mL in PHGII medium, 10% (v/v) of the suspension was taken to inoculate the two experimental sets in duplicate PH-GII medium and PHGII (-glu) medium. The spent catalyst was added at 8% (w/v) of pulp density, and a negative control (non-inoculated) was also set up to assess abiotic metal removal. After 7 days of incubation at 30 °C and 150 rpm, microbial growth was determined by serial dilutions and viable counts, and the liquid phase was filtered using a cellulose acetate syringe filter (Alltech, Deerfield, IL, USA). The filtrate was placed in a 40 mL glass tube and pH was determined in duplicate according to the NMX-AA-008-SCFI-2011 (DOF, 2012) method, using a digital potentiometer (PerpHect LogR meter 310). Biologically treated spent catalyst was dried at room temperature for 48 h, and Ni and V residual concentrations were determined at the beginning and at the end of experimentation by Inductively Coupled Plasma Optical Emission Spectrometer (ICP-OES), as mentioned below. Data of Ni and V removal corresponded to biological activity, since results of abiotic removal were subtracted before reporting data.
Digestion of samples and metal analysis
Dry spent catalyst 100 mg samples were placed in silicon carbide cylindrical vials and digested with HNO3 6 mL and HCl 2 mL in a Multi-wave PRO Microwave Reactions System (Anton Paar), using the HF100 rotor. Digestion conditions were: power 800 W for eight vessels, 40 bars, temperature 210 °C - 240 °C, pRate 0.3 bar seg-1, ramp 15 min, hold 30 min and cooling 15 min. Afterwards, 20 mL of deionized water was added to each cylindrical vial, and the supernatant was collected in a 100 mL volumetric flask to set the volume with deionized water. After acid digestion, catalyst samples were subjected to Ni and V metal analyses using ICP-OES (Varian Model 710-ES) at the following wavelengths (nm): Ni (231.604) and V (292.401). Ni and V residual concentrations were calculated based on a calibration curve of 0.1 ppm - 10 ppm using a commercial standard (High-Purity) Cat. # ICP-200.7-6.
Results
Microbial growth in presence of the spent catalyst
Previous determination of metal content in H-oil spent catalyst showed the presence of Ni and V at 24 822 mg/kg and 57 617 mg/kg, respectively, along with other metals like As, Cr, Cu, Fe, Mg, Mo, P, Al, Zn, and 36 % (w/v) of oil hydrocarbons (Gómez-Ramírez et al., 2014). Then, to evaluate the ability of the three Bacillus spp. strains coded as MV-9K-2, MV-AH-1, and MNSH1-9K-1 to grow in the presence of the spent catalyst at 8 % (w/v) of pulp density, and to evaluate the growth changes that may occur in the presence or absence of glucose, each strain was grown in PHGII liquid medium or PHGII (- glu), as described in Materials and Methods. Results showed that after 7 days of incubation, strain MNSH1-9K-1 presented a significant decrease in cell viability compared to the initial cell concentration of 0.4-fold and 0.3-fold in PHGII and PHGII (- glu), respectively, and MV-AH-1 also showed a 0.6-fold decrement in both cases (Figure 1). In the other hand, strain MV-9K-2 did not present a statistically significant difference at the end of growth.

Source: Author's own elaboration.
Figure 1 Microbial growth on spent catalyst at 8% (w/v) of pulp density after 7 days of incubation at 30 °C and 150 rpm in PHGII medium or PHGII (- glu). Data are presented as averages (n = 2) ± standard deviations, and lowercase letters indicate groups of data that were not significantly different by Analysis of Variance (ANOVA) (p > 0.05).
Changes in pH values during metal removal from spent catalyst
It has been reported that, under acidic conditions, heavy metals may present higher toxic effects than at neutral pH values, since the proportions of toxic free ionic forms of the metals increase with pH lowering (Pennanen, 2001). In this regard, initial pH of abiotic control decreased from 7 to 4.010 ± 0.000 when the spent catalyst was added at the beginning of both experiments at 0.2% (w/v), caused by the high metal content of the spent catalyst (Table 1). Likewise, in PHGII medium and PHGII (- glu), pH decreased between 3.690 and 3.855 when the spent catalyst was added in the three culture experimental sets (with no significant differences between them). After 7 days of incubation, further pH diminutions were differentially observed. While the controls (without cell inoculation) showed a final pH raging from 2.165 to 2.205 due to abiotic lixiviation, cultures from all the isolates showed significantly different pH final values in the ranges: 2.510 ± 0.170 for MV-9K-2, 2.710 ± 0.042 for MNSHI-9K-1, and 2.250 ± 0.042 for MV-AH-1. Furthermore, no significant pH differences were observed in PHGII medium.
Table 1 Initial and final pH values reported during metal removal from the spent catalyst
TO | T7 | ||
---|---|---|---|
Strain | PHGII; PHGII (- glu) | PHGII | PHGII (- glu) |
MNSHI-9K-1 | 3.785 ± 0.063 a | 2.675 ± 0.007 c | 2.710 ± 0.042 c |
MV-AH-1 | 3.855 ± 0.063 a | 2.260 ± 0.000 d | 2.250 ± 0.042 d |
MV-9K-2 | 3.690 ± 0.056 a | 2.615 ± 0.007 e | 2.510 ± 0.170 e |
Abiotic Control | 4.010 ± 0.000 b | 2.205 ± 0.021 d | 2.165 ± 0.021 d |
a - e, lowercase letters indicate groups of data that were not significantly different by ANOVA (p > 0.05).
Source: Author's own elaboration.
Metal removal from spent catalyst
Results of Ni and V removal from spent catalyst at 8 % (w/v) pulp density in PHGII medium revealed that the three isolates MNSH1-9K-1, MV-AH-1, and MV-9K-2 were able to remove 913.87 mg/kg ± 55.99 mg/kg, 1164.37 mg/kg ± 38.74 mg/kg and 2541.70 mg/kg ± 33.07 mg/kg of Ni from spent catalyst when PHGII medium was not added with glucose (PHGII glu (-)), and their Ni removal capabilities significantly decreased in a 99.35%, 78.19%, and 56.36% in PHGII medium, respectively, showing Ni removals of 5.88 mg/kg ± 0.32 mg/kg, 253.89 mg/kg ± 3.54 mg/kg and 1109.29 mg/kg ± 32.28 mg/kg (Figure 2). Thus, the strain MV-9K-2 presented the highest Ni removal ability, which corresponds to 1109.29 mg/kg ± 32.28 mg/kg and 2541.70 mg/kg ± 33.07 mg/kg in PHGII (- glu) medium. On the other hand, only two isolates MNSH1-9K-1 and MV-9K-2 were capable of removing V from the spent catalyst. The first strain was able to remove 990.26 mg/kg ± 27.85 mg/kg in PHGII medium, being the latter strain the one that showed the highest removal value again, corresponding to 3701.28 mg/kg ± 62.37 mg/kg in PHGII (- glu) medium (Figure 3).
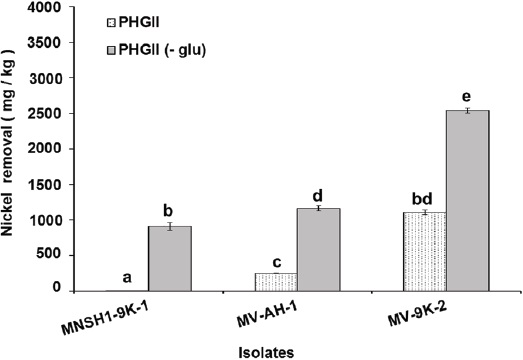
Source: Author's own elaboration.
Figure 2 Nickel removal from spent catalyst at 8 % (w/v) of pulp density in PHGII medium and PHGII (-glu) medium, the presence or absence of glucose after 7 days of incubation at 30 °C and 150 rpm in PHGII medium. Data are presented as averages (n = 2) ± standard deviations, and lowercase letters indicate groups of data that were not significantly different by ANOVA (p > 0.05).

Source: Author's own elaboration.
Figure 3 Vanadium removal from spent catalyst at 8 % (w/v) of pulp density in PHGII medium and PHGII (-glu) medium after 7 days of incubation at 30 °C and 150 rpm in PHGII medium. Data are presented as averages (n = 2) ± and standard deviations, and lowercase letters indicate groups of data that were not significantly different by ANOVA (p > 0.05).
Discussion
Previous reports with Bacillus megaterium MNSH1-9K-1 correlated the different growth phases with the metal removal capability and was observed that the maximum removals of Ni and V were achieved when bacterial strains were in the stationary phase of growth (Fierros-Romero, Rivas-Castillo, Gomez-Ramírez, Pless & Rojas-Avelizapa, 2016b). In the present study, for the three isolates evaluated, the same growth yields were observed in both systems after 7 days: PHGII medium and PHGII (- glu) medium, suggesting that growth in the presence of the spent catalyst is not affected by glucose. B. megaterium is a microorganism that has been reported as heterotrophic bacteria, but there are also some studies which report its mixotrophic capacity, the ability that can be develop depending on the environment to which the microorganism is exposed, being able to grow without an organic carbon source such as glucose (Dong & Reddy, 2010). The growth differences between the three strains could be attributed to each isolate resistance capability to the spent catalyst, as shown in the significant viability differences at the end of the experiment. Recently, diverse molecular resistance mechanisms of Bacillus megaterium during metal removal from a spent catalyst are reported, and they showed evidence of a differential set of molecular mechanisms that may provide B. megaterium strain with a clear advantage to survive metal stress. It was demonstrated that rpoS encodes a sigma factor, which is a central regulator of the general stress response, allowing the cells to survive environmental challenges and preparing the cell for subsequent stresses. It is possible that an enhanced expression of molecular mechanisms, such as the alternative sigma factor rpoS and other relevant Ni-V uptake mechanisms, may be present in B. megaterium MNSH1-9K-1 during exponential growth and other growth phases, conferring this strain with heightened metal resistance and removal abilities (Rivas-Castillo, Orona-Tamayo, Gómez-Ramírez & Rojas-Avelizapa, 2017). This is in accordance with previous reports which show that there could be factors considered to affect microbial growth in the presence of a spent catalyst, as: a) composition of the spent catalyst, b) microorganism type, c) population density, d) strain metal tolerance, and e) adaptation abilities of each microorganism (Pradhan, Nathsarma, Srinivasa, Sukla & Mishra, 2008).
Although it has been observed that carbon sources can differentially affect the biomass production of B. megaterium (Santos, Nego, Manchado, Soares & Soares, 2013), it has been also suggested that the presence of heavy metals may interfere with glucose internalization by the cells (Stoll & Duncan, 1996) similar to the action of metal uncouplers (Borst-Pauwels, 1981); thus, preventing the carbon source influence on culture growth.
Acidification of media may be expected when adding the spent catalyst, as it has been stated that pH values and heavy metal concentrations are closely related (Pennanen, 2001). Bacteria express a wide range of complex molecules on their cell wall, which confer anionic net charge to the cell surface at acid pH values (Vieira & Volesky, 2000). When the cell wall is in direct contact with the environment, negatively charged groups can attract and bind metallic cations based on electrostatic forces without cellular energy consumption, an effect that is favored by the high surface-volume ratio in bacteria (Rodríguez, Quesada & Rodríguez, 2006). Also, previous studies have shown that modifications in the environmental conditions such as pH, temperature, and electron donor availability can alter the trans-membrane pH gradient, the total proton motive force, and affect the internal pH of the cells, as well as their energetics (Repaske & Adler, 1981). When these changes are present, cells need to adapt for surviving (Srinivasan & Mahadevan, 2010), and in order to maintain energy homeostasis, cells have to actively regulate the internal pH by secreting or consuming protons, as it has been predicted by in-silico models constructed to describe the intracellular metabolism changes for organisms such as E. coli and B. subtilis across a wide range of growth environments (Feist & Palsson, 2008; Palsson, 2000; Reed & Palsson, 2003; 2004; Oh, Palsson, Park, Schillin & Mahadevan, 2007). Thus, the results suggest that pH differences are glucose-independent under the conditions tested. This may probably be attributed to each strain metabolic response to the spent catalyst, or perhaps due to the affectation of the kinetics and the thermodynamic feasibility of biochemical reactions for active pH regulation; which can have a direct impact in the cell viability and in the biomass yield of an organism, as it has been previously reported for microorganisms like E. coli (Srinivasan & Mahadevan, 2010).
It has been previously reported that medium composition has a remarkable effect on microbial-cell wall composition and certain cell wall properties, such as the type of polar groups present and the charge within the cell wall macromolecules, which may influence the cell affinity to specific metals (El-Sersy & El-Sharounty, 2007). In Saccharomyces cerevisiae, the mechanism of inhibition by 2-de-oxy-glucose of the synthesis of yeast wall polysaccharides and glycoproteins was investigated, and they showed that the extent of the inhibition of manna and glucan synthesis was found to be dependent on whether glucose or man-nose was used as the carbon source in the medium (Krátký, Biely & Bauer, 1975). Besides, Hassan & El-Kassas (2012) studied medium compositions for Cd uptake by marine Aspergillus cristatus and showed that the main factors having negative effects on metal uptake was glucose. Thus, the results show that adaptability and metal removal capabilities differ between the strains MNSHI-9K-1, MV-9K-2, and MV-AH-1, and that each strain possesses specific abilities that may have been differentially developed due to previous adaptations attained in-situ, as they were isolated from high metal content mining sites (Gómez-Ramírez et al., 2012). Recently, Bacillus megaterium strain MNSH1-9K-1 shows the ability to remove Ni and V from spent petrochemical catalysts containing 428 mg/kg ± 30 mg/kg of Ni and 2165 mg/kg ± 77 mg/kg of V, and the metal uptake genes nccA, hant, VAN2, and smtAB were detected by PCR (Fierros-Romero, Gómez-Ramírez, Arenas-Isaac & Pless 2016a). Also, there were changes detected in the expression of the genes nccA (Ni-Co-Cd resistance); hant (high-affiinity nickel transporter); smtAB, a metal-binding protein gene; and VAN2 (V resistance) after the microorganism exposure to 200 ppm of Ni and 200 ppm of V during the stationary phase in PHGII liquid medium (Fierros-Romero et al., 2016b). The literature reports several studies on the interaction of heavy metals with bacterial surfaces, but just few works consider these interactions at a molecular level. Even more, the presence of a carbon source caused strain-dependent effects on Ni and V removal from the spent catalyst, as previously reported for metal uptake as-says with Pseudomonas aeruginosa (Sar et al., 1998) and Aspergillus cristatus (Hassan & El-Kassas, 2012). These observed differences may be possibly related to: 1) cell wall properties, as the presence of specific anionic polymers (Öztürk, 2007), whose metal interaction capability may be altered by the presence or absence of glucose, or 2) distinctive metabolic glucose-dependent and independent, signaling pathways that may confer each strain with diverse resistance mechanisms and metal uptake patterns. Rivas-Castillo et al. (2017) investigated the resistance of B. megaterium MNSH1-9K-1 to H-oil spent catalyst and compared it to the resistance of the type strain QMB1551. Both strains were cultivated at 37 °C in liquid LB medium containing different concentrations of the spent catalyst. After 24 h of exposure to the catalyst, cell viability was determined, showing that the isolated MNSH1-9K-1 strain presents a clear growth advantage in the presence of H-oil, compared to the resistance shown by the type strain QMB1551. Also, proteomes were further analyzed by 2-DE, and the patterns confirmed clear differences between the proteomic patterns of the two B. megaterium strains when grown in presence or absence of the spent catalyst (Rivas-Castillo et al., 2017).
Conclusions
In conclusion, the current study showed that Bacillus megaterium strain MV-9K-2 presented the best Ni and V removal ability from the spent catalyst, corresponding to 2542 for Ni and 3701 mg/kg for V, exhibiting the biotechnological potential of Bacillus spp., especially B. megaterium MV-9K-2, for spent catalysts biotreatment. Although further studies are needed to elucidate the mechanisms involved in this Ni and V uptake, the results strongly suggest that the presence of glucose, as in PHGII medium, may affect B. megaterium metal removal capability from spent catalysts.