INTRODUCTION
Quantum dots (QDs), approximately 2-100 nm in diameter, are luminescent semiconductor nanocrystals. QDs have advanced optical properties compared with traditional organic fluorophores: (i) high brightness due to the extinction coefficient and quantum yield, (ii) broad absorption characteristics and a narrow line width in emission spectra, (iii) continuous and tunable emission maxima due to quantum size effects and (iv) longer fluorescence lifetime ranging from 10 to 40 ns. Their controllable tiny size (in nanoscale) gives QDs good biocompatibility; some QDs can easily pass biological barriers such as cell membranes (Jaiswal et al. 2003).
Quantum dots (QDs) have been widely studied as absorbers for various solar technologies because of their excellent optoelectronic properties, such as a size-dependent absorption spectrum, efficient charge separation and transport, and good photostability. During the last decade, major research initiatives have been pursued to elucidate the structure-dominated optoelectronic properties with the goal of maximizing overall solar-device power-conversion efficiency (Zhao et al. 2017). Semiconductor nanocrystals or quantum dots (QDs) that are composed by elements such as Cd, Te, Se, Pb and As, among others, exhibit unique optical and electronic properties with fluorescence emission wavelengths depending on particle size (Michalet et al. 2005, Zhou and Ghosh 2007, Fulekar 2012). The As-prepared QDs exhibit high fluorescence intensity, excitation-dependent photoluminescence behavior and bright blue fluorescence under ultraviolet lamp (Yan et al. 2016). With the increasing application of semiconductor particles, especially metal-based QDs, these quantum dots will inevitably be released into the environment, and therefore their effects on biota should be assessed. Few studies on the effects of QDs on microorganisms and phytoplankton have been performed (Yan et al. 2015a). A novel sensing system has been designed for the detection of m-phenylenediamine based on carbon QDs improved chemiluminescence in a luminol-potassium permanganate (KMnO4) system. The carbon QDs were synthesized by pyrolyzing a mixture of citric acid and glycine. Under the action of carbon QDs and KMnO4, the luminol can be oxidized by synergy, which significantly increases the chemiluminescence intensity (Yan et al. 2015b). The carbon QDs, as a low cost, chemically stable, and environmentally friendly photosensitizer, can dramatically broaden the light absorption range to the entire visible range (Ye et al. 2017).
QDs are also excellent probes for two-photon confocal microscopy because they are characterized by a very large absorption cross section (Larson et al. 2003).
QDs are heterogeneous nanoparticles that consist of a colloidal core surrounded by one or more surface coatings. Surface coatings in one or more layers are frequently applied to customize QDs to specific applications, such as the use of hydrophilic coatings to increase solubility in a biologically compatible medium, coatings (or ‘‘shells’’) that reduce leaching of metals from the core (Derfus et al. 2004).
QDs are bright, photostable fluorophores that have a broad excitation spectrum but a narrow Gaussian emission at wavelengths controllable by the size of the material. QDs allow for efficient multicolor imaging of biological samples (Chan et al. 2002) and should be especially useful for fluorescence imaging in living tissues, where signals can be obscured by scattering and competing intrinsic emissions. Multiphoton microscopy enables deep imaging of a variety of biological samples with less overall photobleaching than with wide-field or confocal microscopy, and it has now become the primary fluorescence imaging technique in thick specimens (Denk et al. 1990, Williams et al. 2001).
QDs are materials with the core/shell that contain a core of a material such as cadmium selenide, and a cover such as zinc sulfide (Malik et al. 2002).
It is clear that the rapid growth of interest in engineered nanoparticles has presented many challenges for ecotoxicology, not least being the effort required to analyze and understand the nanoparticles themselves. A considerable amount of progress has been made in understanding the fate of nanoparticles in porous media and a limited understanding of the fate of nanoparticles in surface waters is being developed. It seems likely that, although the nanoparticles themselves are complex systems, a reasonable understanding of their fate and behavior in the natural environment may be developed (Christian et al. 2008).
QDs are a very good model to determine the penetration of nanoparticles in skin. For such models, QD potential for toxicity and interactions within biological systems must be determined before nanomaterial risk assessments can be made. For imaging and optical sectioning, confocal laser scanning microscopy can precisely localize the QD (or other appropriate fluorophore-conjugated nanoparticles) within the optical sections to localize the nanoparticles in the different skin layers (Ryman-Rasmussen et al. 2006).
The sustainable use of nanomaterials in medical, electronic and environmental applications is very important to understand the interactions of microorganisms in ecosystems, playing an important role in primary productivity and biogeochemical cycles (Mahendra et al. 2008).
The technological advances associated with nanomaterials and their many applications have resulted in the introduction of metal contaminants in the form of nanoparticles with unknown consequences to natural ecosystems. Understanding the physical and ecotoxicological properties of nanoparticles in natural aquatic systems is critical for developing adequate regulatory structures to protect aquatic ecosystems from their detrimental effects. An increasing number of studies have demonstrated both sub-lethal and toxic effects of engineered nanoparticles to bacteria, (Mahendra et al. 2008, Fabrega et al. 2009, Muhling et al. 2009, Slaveykova et al. 2009) invertebrates (Bouldin et al. 2008, Ward and Kach 2009) fish (Wong et al. 2010) and mammals (Geys et al. 2008).
Attention has been devoted to the toxicology (Oberdörster et al. 2005, Lam et al. 2006, Nel et al. 2006) and health (Kreyling et al. 2006, Helland et al. 2007) implications of nanoparticles, while the environmental behavior of engineered nanoparticles has been less studied (Biswas and Wu 2005, Wiesner et al. 2006, Helland et al. 2007).
In aquatic risk assessment, algal growth inhibition assay is widely used (Radix et al. 2000, Blinova 2004). Pseudokirchneriella subcapitata (formerly known as Selenastrum capricornutum and Rhapidocelis subcapitata) is considered a model organism for freshwater algae and is widely used in the algal growth inhibition test (OECD 1984) as well as in the US-EPA green algae growth inhibition test (US-EPA 1996). This species has also proven to be very sensitive to heavy metals (Blinova 2004, Kahru et al. 2005). However, only few studies have investigated nanoparticle toxicity to algae (Aruoja et al. 2009).
Because the use of the QDs is increasing, it is necessary to perform essays for establishing their toxicity. Therefore, the aim of the present paper is to assess the ecotoxicological effects of different QDs on the microalgae P. subcapitata. We have evaluated the ecotoxicity effects of three kinds of QDs (QDs[a] for orange fluorescence; QDs[r] for red fluorescence and QDs[v] for green fluorescence) using P. subcapitata as a model organism. Through determination of the algal growth inhibition (IC50) as a response to acute toxicity tests, we can describe the impacts of QDs on P. subcapitata. This is the first report on the toxicity evaluation of the three kinds of QDs on fresh water algae at the same time, and further comparison of the toxicity of different QDs.
MATERIALS AND METHODS
Culture of Pseudokirchneriella subcapitata
The microalgae P. subcapitata was obtained from the Centro Nacional del Medio Ambiente (National Center for the Environment, CENMA) of the Univer- sity of Chile. The microalgae were grown in Oligo L.C. medium without EDTA (AFNOR 1980). Culture media were autoclaved at 121 ºC for 15 min. Micro- algal cells were cultured in 1 L of medium in 2 L borosilicate Erlenmeyer flasks under 12 h : 12 h light/dark periodic lighting intensity of 100 μmol photons m2/s provided by fluorescent light (Fluora; Osram) with controlled temperature (24 ± 2 ºC)
Ecotoxicological bioassays
The concentration of QDs in the standard solution was 4 M, from which the following solutions: 10-1 M, 10-2 M, 10-3 M, 10-4 M, 10-5 M, 10-6 M, 10-7 M, 4 nM, 3 nM, 2 nM, and 1 nM, were prepared with orange, red and green fluorescence (Fig. 1). The ecotoxicological bioassays of P. subcapitata were exposed for 96 h to concentrations of 0, 1, 2, 3 and 4 nM QD nanoparticles based on the protocol of the US Environmental Protection Agency (US-EPA 2002) and other protocols such as ISO 14669:199E (ISO 1999), ISO 10253:2006 (ISO 2006) and the one developed by Gorbi et al. (2012). Each concentration was replicated three times in 1500 μL polypropylene microcentrifuge tubes containing 200 μL of medium at different concentrations of QDs. P. subcapitata cells in the exponential growth phase were inoculated into the microcentrifuge tubes providing initial cell densities of approximately 104 cells/mL. The microcentrifuge tubes were maintained on an orbital shaker at 150 rpm. The environmental conditions used for the experi- ments were described based on cultures of microalgae (ABNT 2005). After sufficient pre-experiments, the initial cell density of algae was set to 1 × 104 cell/mL. Three replicates were performed for each concentration. The P. subcapitata growth inhibition rate was one of the indices of the QDs ecotoxicity. At the end of the 96 h period the inhibition of growth of P. subcapitata was evaluated, determining the toxicity, which produces a toxic response at a level of 50 %, as the mean inhibitory concentration (IC50) that inhibits the growth of 50 % of the population of P. subcapitata.
Fluorescence of P. subcapitata impregnated with QD nanoparticles
The highly fluorescent CdS QD nanoparticles were obtained from the Laboratorio de Nanotecnología y Microbiología (Bionanotechnology and Microbiology Laboratory) of the Andrés Bello National University (Santiago, Chile). The fluorescence spectra were determined in samples of different concentrations of QD nanoparticles with cultures of the microalgae P. subcapitata using an ultraviolet (UV) lamp with CAMAG® UV Lamp 4 dual wavelength 254/366 nm, 2 x 8 W (Fig. 1).
Fluorescence confocal microscopy for P. subcapitata impregnated with QD nanoparticles
Samples of the microalgae P. subcapitata attached to QD nanoparticles at different concentrations were mounted on slides with fixative (DAKO North America Inc., S3023) to visualize them in a Leica TCS-SP8 spectral fluorescence confocal microscope (wavelength: 425-520 nm), with the Leica confocal software (Leica Microsystems) (Figs. 2, 3).

Fig. 2 3D images in fluorescence confocal microscopy of the P. subcapitata microalgae impregnated with quantum dot nanoparticles
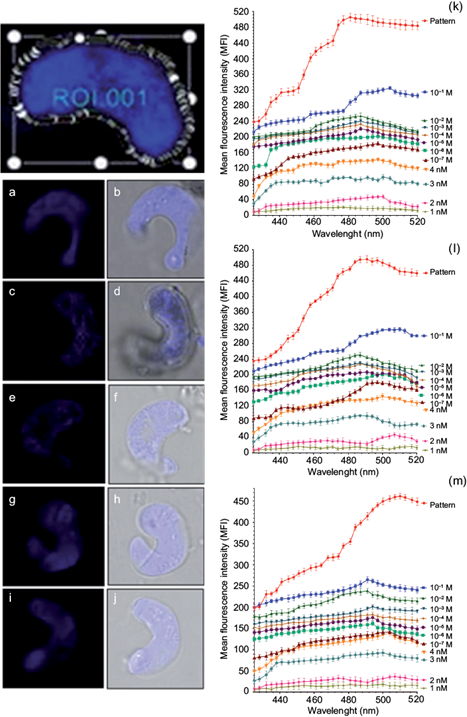
Fig. 3 Fluorescence confocal microscopy images and scans of the microalgae P. subcapitata impregnated with quantum dot nanoparticles at different concentrations. (a) Fluorescence image (FI) of P. subcapitata in a standard solution of red fluorescence. (b) Phase contrast image (FCI) of P. subcapitata in a standard solution of red fluorescence. (c) FI of P. subcapitata in a 10-1 M solution of orange fluorescence. (d) FCI of P. subcapitata in a 10-1 M solution of orange fluorescence. (e) FI of P. subcapitata in a 10-2 M solution of green fluorescence. (f) FCI of P. subcapitata in a 10-2 M solution of green fluorescence. (g) FI of P. subcapitata in a 10-3 M solution of green fluorescence. (h) FCI of P. subcapitata in a 10-3 M solution of green fluorescence. (i) FI of P. subcapitata in a 10-3 M solution of red fluorescence. (j) FCI of P. subcapitata in a 10-3 M solution of red fluorescence. Representation of the maximum and minimum fluorescence intensities of P. subcapitata at different concentrations of quantum dots with (k) orange fluorescence, (l) red fluorescence, (m) green fluorescence.
Statistic analysis
The log-normal model was used with the software REGTOX_EV7.0.6 for Microsoft Excel (Vindimian 2014), in order to calculate the IC50 of P. subcapitata microalgae following the ISO/TS 20281 procedure (ISO/TS 2006). Analysis of variance (ANOVA) was used to determine the statistical significance of differences between values.
RESULTS
Fluorescence of cultures of P. subcapitata attached to quantum dot nanoparticles
Reference toxins such as QD nanoparticles adhered to the P. subcapitata microalgae exhibit different fluorescence such as orange, red and green when they are exposed to the ultraviolet light observed in the standard solution (4M) of QDs and 10-1 M. The green fluorescence was not observed at the 10-1 M concentration alone (Fig. 1).
Sensitivity of P. subcapitata to quantum dot nanoparticles determined by confocal fluorescence microscopy
Figure 2 shows the three-dimensional structure of the P. subcapitata cell in a 3D confocal microscopy image with the following measurements: 8 μm on the x and y axes, and 6 μm in the z axis (depth), where it is observed in different colorations.
Figure 3 shows images and scans in confocal fluorescence microscopy of P. subcapitata impregnated with QD nanoparticles at different concentrations. Panels (a), (c), (e), (g), and (i) in figure 3 show fluorescence images of P. subcapitata in the QDs standard solution with red fluorescence, in the 10-1 M solution with orange fluorescence, in the 10-2 M solution with green fluorescence, in the 10-3 M solution with green fluorescence, and in the 10-3 M solution with red fluorescence. Figure 3b,d,f,h,j shows phase contrast images of P. subcapitata. in the standard solution with red fluorescence, in the 10-1 M solution with orange fluorescence, in the 10-2 M solution with green fluorescence, in the 10-3 M solution with green fluorescence, and in the 10-3 M solution with red fluorescence.
Figure 3k,l,m shows the maximum peak of fluorescence in the standard solution, corresponding to 481 nm in QDs with orange fluorescence, 491 nm in QDs with red fluorescence, and 510 nm in QDs with green fluorescence. The results demonstrate that the peak of fluorescence decreases to different wavelengths. In this study a decrease was observed in the curves of mean intensity of fluorescence (MIF) from the 4 M standard solution until1 nM of QDs, as shown below:
(a) Orange, red and green fluorescence at different concentrations of QD nanoparticles detected in microalgal cells of P. subcapitata correspond to an optical section xyz shown in figure 3k,l,m. The results show that the mean intensity of fluorescence (MIF) decreases while the concentration of QDs decreases from 10-1 to 10-9 M, finding statistically significant differences (p < 0.05).
(b) Regarding the orange fluorescence in the microalgal cell P. subcapitata at different concentrations of QD nanoparticles, the mean intensity of fluorescence (MIF) decreases with the following values: 10-1 M, MIF = 325; 10-2 M, MIF = 254; 10-3 M, MIF = 241; 10-4 M, MIF = 233; 10-5 M, MIF = 221; 10-6 M, MIF =2 02; 10-7 M, MIF = 184; 4 nM, MIF= 140; 3 nM, MIF = 97; 2 nM MIF = 48, and 1 nM, MIF = 21 (Fig. 3k).
(c) Concerning the red fluorescence in P. subcapitata at different concentrations of QD nanoparticles, MIF decreases with the following values: 10-1 M, MIF = 316; 10-2 M, MIF = 251; 10-3 M, MIF = 230; 10-4 M, MIF = 2º29; 10-5 M, MIF = 207; 10-6 M, MIF = 201; 10-7 M, MIF = 180; 4 nM, MIF = 145; 3 nM, MIF = 96; 2 nM, MIF = 47; and 1 nM, MIF = 17 (Fig. 3i).
(d) With respect to the green fluorescence in P. subcapitata at different concentrations of QD nanoparticles, the mean intensity of fluorescence MIF decreases with the following values: 10-1 M, MIF = 265; 10-2 M, MIF = 239; 10-3 M, MIF = 201; 10-4 M, MIF = 204; 10-5 M, MIF = 176; 10-6 M, MIF = 162; 10-7 M, MIF = 142; 4 nM, MIF = 139; 3 nM, MIF = 93; 2 nM, MIF = 37; and 1 nM, MIF = 19 (Fig. 3m).
Inhibitory concentration of growth of P. subcapitata with QD nanoparticles
The exposure of P. subcapitata to different concentrations (0, 1, 2, 3, and 4 nM) of QD nanoparticles caused an IC50 in the microalgae of 1.94 nM of QDs with orange fluorescence, of 1.68 nM with red fluorescence and of 2.03 nM of with green fluorescence at 96 h of exposure (Fig. 4a, b, c).
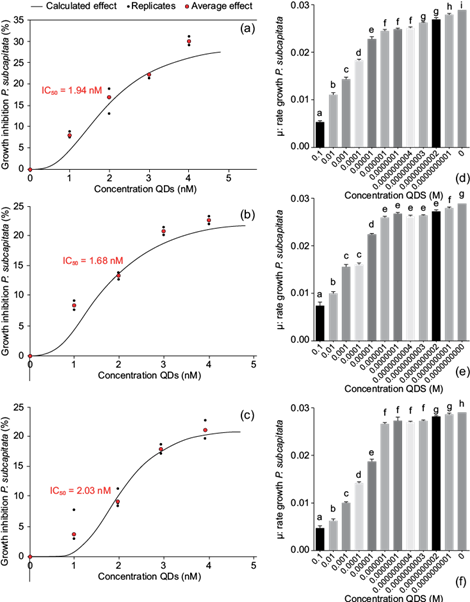
Fig. 4 Inhibitory growth concentrations (IC50) of P. subcapitata at different concentrations of quantum dot nanoparticles during a 96-h exposure: (a) orange fluorescence, (b) red fluorescence, and (c) green fluorescence. (d), (e), (f) Growth rate of P. subcapitata at different QDs concentrations with orange, red, and green fluorescence, respectively
As for the bioassays, a growth rate (μ) of P. subcapitata was observed in the different concentrations of orange, red and green fluorescence, being higher in 1 nM (μ = 0.028, QDs with orange fluorescence; μ = 0.027, QDs with red fluorescence; μ = 0.028, QDs with green fluorescence). The growth rate decreased from 1 nM to 10-1 M QDs with orange fluorescence nanoparticles (0.028 to 0.0053), with red fluorescence (0.027 to 0.0073) and with green fluorescence (0.028 to 0.0046) (Fig. 4d, e, f).
DISCUSSION
The fluorescence of P. subcapitata with QD nanoparticles was observed in the 4 M and 10-1 M standard solution of QDs when it was excited by ultraviolet light emitting orange, red and green fluorescence, which is consistent with the observations of Gallardo et al. (2014) in their study about the effects of phosphate and citrate on the production of QDs, in which they evaluate the determination of fluorescence (green, red and orange) of the Pseudomonas isolates.
Lin et al. (2009) also reported the adsorption of QDs to algal cells increased by the dosage of QDs, and mentioned that the chemical composition of the porous algae cell wall consists of cellulose, polysaccharides, and glycoproteins, which afforded numerous QDs binding sites through nonspecific (electrostatic, hydrophobic, and hydrogen bonding) interactions. The binding affinity of QDs to algae can also be due to the interaction between carboxylic (-COOH) and amine (-NH2) groups of the wall of the algal cell. The surface of a typical algal cell (~10 µm in diameter) is at least 250 000 times larger than that of a QD (< 20 nm in diameter), which allowed for a significant amount of the QDs to be adsorbed (Lin et al. 2009). The IC50 value (mg/L) of QDs was determined with a biotest growth inhibitor (algal cells counting) shown in Fig. 4.
Xiao et al. (2016) used Chlorella pyrenoidosa as a model organism to evaluate the potential environmental risks of QDs. To the best of our knowledge, it is the first report of the toxicity evaluation of six kinds of QDs on freshwater algae at the same time and the toxicity of different QDs can be compared using the same parameters. C. Pyrenoidosa was treated with various concentrations of QDs. According the IC50 of six QDs, their toxicity order was: CuInS2/ZnS QDs < no doped carbon QDs (CCQDs) < nitrogen doped CQDs < nitrogen, sulfur doped CQDs < CdS QDs < CdTe QDs. It was demonstrated that the toxicity of three kinds of CQDs was lower than MQDs except for CuIn S2/ZnS QDs, whose toxicity was not only compared with carbon dots for the first time, but also its ecotoxicity on an aquatic organism was firstly investigated.
Xiao et al. (2016) found that during 144 h (6 days), the algae density of C. Pyrenoidosa almost equaled that of the control group at low concentrations of QDs. However, at high concentrations, QDs produced negative impacts on the algal growth and inhibited the cells growth significantly.
In another study, Bouldin et al. (2008) showed that QD nanoparticles in P. subcapitata caused an IC50 = 9.638 μg/L with cadmium and an IC50 = 2.410 μg/L with selenium after 96 h of exposure.
According to Jackson et al. (2012) in a study on the bioavailability, toxicity and bioaccumulation of QDs nanoparticles (CdSe/ZnS) ingested by algae for food of amphipods and QDs in aqueous medium exposed to amphipods, resulting in high amphipod mortality exposed to algae that ingested QDs nanoparticles showing to be bioavailable with higher toxicity (IC50 = 0.61 nM) in comparation with amphipods exposed to an aqueous medium with QDs nanoparticles with toxicity (IC50 = 2.93 nM) not reaching 50% of mortality.
According to Jackson et al. (2012) in a study on the bioavailability, toxicity and bioaccumulation of QD nanoparticles (CdSe/ZnS) ingested by algae food for amphipods in an aqueous medium, this exposure resulted in high amphipod mortality when QDs were bioavailable with higher toxicity (IC50 = 0.61 nM) in comparison to amphipods exposed to an aqueous medium with QDs nanoparticles with lower toxicity (IC50 = 2.93 nM), not reaching 50 % of mortality.
Being QDs very dangerous, Ryman-Rasmussen et al. (2006) studied the cellular flow diffusion of this nanoparticles when applied topically to the porcine skin. They demonstrated with confocal microscopy the penetration of QDs in the stratum corneum located inside the epidermis and dermal layers, and their ability to penetrate intact skin in the lapse of a working day (8 h exposure), concluding that the skin is surprisingly permeable to nanomaterials. A study by Bouldin et al. (2008) about QD nanoparticles with organic coating adhered on P. subcapitata, resulted in an IC50 = 67 nM, providing evidence that the protective coating of carboxyl allowed for cell protection during the adsorption.
The toxicity mechanisms of QDs on algae are: (1) QDs induce cells to produce reactive oxygen species (ROS), and cells suffer oxidative damage (Finkel and Holbrook 2000); (2) QDs release their core metals into water (Klaine et al. 2008); (3) some QDs have surface modifications, and toxicity depends on the substances attached to their surface (Hoshino et al. 2004). Metallic QDs may release their core metals into water, and the released metal ions are toxic to aquatic organisms even at a relatively low concentration. The toxicity of CdTe QDs and CdS QDs is attributed to the core material, Cd. The detailed synthesis procedures of CdTe QDs and CdS QDs are different, and the elements introduced by the synthesis are not the same, so their toxicity may be significantly different. Wang et al. (2016) also reported low toxicity of TiO2 nanoparticles to P. tricornutum, which exhibited a significant growth inhibition at nanoparticle concentrations higher than 20 mg/L and 120 h of exposure, with half maximal effective concentration (EC50) of 167 mg/L.
Growth inhibition was also reported in the marine diatom Nitzschia closterium exposed to TiO2 NPs, but only at very high concentrations (EC50 of 89-119 mg/L) for 96 h (Xia et al. 2015). Furthermore, our data are roughly in agreement with Aruoja et al. (2009), who reported the toxicities of bulk and ZnO nanoparticles were both similar to that of ZnSO4 (72 h, EC50 ~0.04 mg Zn/L). On the other hand, Li et al. (2015) reported 72 h EC50 values of 10.7 and 7.4 mg/L of TiO2 NPs for Karenia brevis and Skeletonema costatum, respectively. These contrasting results suggest that NPs toxicity can depend not only on the algal species tested, but also on the type of NPs tested or the different experimental conditions, which include illumination, salinity of culture medium, natural vs. artificial seawater, initial cell density. Moreover, toxic effects of NPs were found in freshwater algae at lower concentrations. In fact, measurable growth inhibition was found for Chlamydomonas reinhardtii at just 0.1 mg/L TiO2 NPs (Chen et al. 2012) and EC50 of only 5.8 mg/L for P. subcapitata (Aruoja et al. 2009). These results can be explained with a lower aggregation process occurring in fresh water compared to sea water, which allow the presence of small aggregates or even dispersed NPs (Handy et al. 2008). Keller et al. (2010) reported that the size of aggregates of TiO2 NPs is about 300 nm in freshwater and 1000-2000 nm in seawater.
Xiao et al. (2016) determined that the EC50 value of three kinds of QDs on C. pyrenoidosa was increased during the exposure time, implying that their inhibition effects declined with increase of exposure time.
CONCLUSIONS
This study contributes to the understanding of predictive ecotoxicology in the impact of QD nanoparticles on aquatic ecosystems, with the following results in the IC50 of P. subcapitata: IC50 = 1.94 nM of QDs (a) for orange fluorescence; IC50 = 1.68 nM of QDs (r) for red fluorescence; IC50 = 2.03 nM of QDs (v) for green fluorescence. The sensitivity of QD nanoparticles bioassays has the following order of sensitivity, being more sensitive the red fluorescence bioassay: QDs (r) ˃ QDs (a) ˃ QDs (v), where the toxicity of QDs is evident by the type of fluorescence stimulated by ultraviolet light with MIF between 140-21 for QDs (a); 145-17 for QDs (r); and 139-19 for QDs (v) at concentrations of 0.1, 2, 3 and 4 nM of QDs. Impregnations of QD nanoparticles observed in very low concentrations as 10-9 M are dangerous since they are permeable to intact skin with different physicochemical properties. In conclusion, these results are of great importance for practical applications of QDs. This work will provide essential data for future studies on the downstream effects of QDs on the microalgae food chain (Fig. 5).