Introduction
Climate is a primary environmental force regulating the establishment and growth of tree seedlings in forest ecosystems (Canham and Murphy, 2016). This makes these early life-cycle stages of trees highly susceptible to climate change (Ibáñez et al., 2007; McLachlan et al., 2007). The latest general circulation models proposed by the Intergovernmental Panel on Climate Change (IPCC) predict a global-scale increase of temperature, while they also anticipate reductions in rainfall across seasonally dry forests of America (Flato et al., 2013). These climatic predictions allow inferring that drought will intensify in these ecosystems, mainly because higher temperatures will increase soil water evaporation and lower rainfall will reduce water inputs (Grossiord et al., 2016). However, little is known yet about how tree recruitment will be affected by these environmental changes.
Tree recruitment in seasonally dry forests occurs during the rainy season (Vieira and Scariot, 2006) and increasing drought due to climate change may negatively affect the regeneration processes that take place during this period. For instance, as several tree species from these ecosystems produce recalcitrant seeds (Zavala-Chávez, 2001; Kettle, 2012), warming may promote seed desiccation and cause the death of embryos, while lower rainfall may reduce the imbibition of seeds and reduce seedling emergence (Walck et al., 2011). Furthermore, seedlings may display negative water balances if warming enhances water loss through transpiration, while reduced rainfall can decrease their capability to compensate these water deficits (Lloret et al., 2004; Ramírez-Valiente et al., 2009). Hence, if the early development of tree seedlings implies elevated water demands, increased drought during rainy season can increase their risk to die because of wilting (Pérez-Ruiz et al., 2018). Nevertheless, plants growing under thermal and/or water stress often display morphoanatomical and physiological shifts addressed to promote their survival under these environmental conditions (Lambers et al., 2008), and this could improve the development and survival of recently emerged tree seedlings under climate change conditions.
Plants exposed to elevated temperatures and/or water shortage conditions usually produce smaller and thicker leaves than conspecific individuals growing under more benign conditions. This reduces foliar heat loading and prevents excessive water loss through transpiration (Quero et al., 2006; Lambers et al., 2008), while it also allows maintaining positive carbon balances because greater amounts of photosynthetic tissues are concentrated on smaller foliar areas (Guerfel et al., 2009; Ramírez-Valiente et al., 2010). Drought stress can also reduce aboveground growth rates of woody plants. This is because the walls of new cells produced by stem meristems quickly lignify and this stops their elongation, which results in shorter aerial shoots with increased wood density (Bouriaud et al., 2005; Moore et al., 2008). Additionally, increased drought often triggers signaling pathways at the whole plant level to promote differential growth of the root over that of the stem in order to prioritize water uptake from the soil (Kozlowski and Pallardy, 2002). Determining whether recently emerged seedlings display this kind of functional shifts when they are exposed to climate change could provide a better understanding about the effects of this phenomenon on the recruitment of tree species from seasonally dry forests.
Oaks (genus Quercus L., family Fagaceae) are conspicuous trees in seasonally dry forests of North America (Nixon, 1993), but several endemic species have constrained distribution ranges because of their highly specific climatic requirements to recruit and survive (Hernández-Quiroz et al., 2018). As these species are more susceptible to climate change than those with wide distribution ranges (McLachlan et al., 2007), this study focused on assessing how the changes in temperature and rainfall predicted to occur during the rainy season may affect the early recruitment of Quercus ariifolia Trel., an oak species endemic to seasonally dry forests of central Mexico. As most oak species, Q. ariifolia produces highly recalcitrant acorns (single-seeded, nut type fruits) that quickly loss viability after they are released from parental trees (Zavala-Chávez and García-Moya, 1996). Hence, we hypothesized that the combination of higher temperature and lower rainfall during the rainy season will promote seed desiccation and reduce seedling emergence in the field. Further, as water shortage quickly promotes wilting in oak seedlings growing in the field (Acácio et al., 2007; González-Salvatierra et al., 2013; Badano et al., 2015), we also hypothesized that seedling survival during the rainy season will be reduced under these climatic conditions. Nevertheless, reductions in survival could be negligible if recently emerged seedlings quickly display morphoanatomical and physiological shifts addressed to face higher temperature and lower rainfall. Otherwise, survival rates of seedling would decrease disproportionately, as compared with those of conspecific individuals growing under the current climate. This study focused on these aims in order to test whether recently emerged seedlings of Q. ariifolia display functional responses addressed to promote their survival under climate change scenarios simulated in the field.
Materials and Methods
Target species
The distribution of Q. ariifolia is concentrated in the Sierra Madre Oriental, Central Mexico (Valencia et al., 2017). Mature trees have coarse leaves covered by thick cuticles, which may be indicative of adaptions to drought. As most oak species, Q. ariifolia has mast reproduction and produces large acorn crops after several years of low or null reproduction. Acorns are developed in summer and they are released from parental trees at the beginning of autumn (Romero-Rangel et al., 2014). Seedling emergence in most oaks from this region starts by the middle of the rainy season (September-October) and seedling establishment mainly occurs beneath the forest canopy (Zavala-Chávez and García-Sánchez, 1999). The development of emerged seedlings extends until January-February, but it fully stops with the start of the dry season in March.
Study site
To assess the effects of changing temperature and rainfall on the early recruitment of Q. ariifolia we performed a field experiment during the rainy season comprised between September 2016 and January 2017. The experiment was located at the wildlife management unit “La Laguna” (21°58'36"N, 100°34'10"W, 2200 m elevation), a private protected area located in the Sierra de Álvarez, westernmost section of Sierra Madre Oriental, state of San Luis Potosí. Mean air temperature in this region is 18 °C, whereas annual rainfall is less than 400 mm in severe dry years and surpasses 600 mm in wet years (Medina-García et al., 2005). Up to 90% of rainfall occur between July and November, but sparse precipitation events can occur until February (Medina-García et al., 2005). Vegetation is composed by primary oak forest, where species are distributed in small monospecific stands that cover less than 1 ha. The most abundant tree species in the study area are Q. ariifolia, Q. mexicana Bonpl., Q. laeta Liebm. and Q. castanea Née.
Climate change predictions
For the study site, we estimated the changes in air temperature and rainfall that will occur during the rainy season (September-January) using the geodatabases of Fernández-Eguiarte et al. (2012; 2014). These geodatabases provide the values of both climatic variables with a spatial resolution of 1 km2 per pixel. Current values are monthly averages that integrate data from 1902 to 2011, while future values are monthly predictions of the HadGEM2-ES general circulation model for year 2040. This model is used to predict the future climate in Mexico because it has lower deviations than other IPCC models when predicting the past climate. These predictions indicate that average air temperature in the study site will increase 1.30-1.64 °C during the rainy season, also predicting increases of 1.07-1.59 °C in maximum air temperature and increases of 0.73-1.27 °C in minimum air temperature. Rainfall, on the other hand, is predicted to decrease 9-21% by the middle of this century (2040-2060). We used these estimates as reference to simulate temperature increases and rainfall reductions in our experiment.
Experimental design
In September 2016, we selected a monospecific stand of Q. ariifolia in the study site and established 20 random points maintaining a minimum distance of 10 m from each other. Half of these points were randomly assigned to controls that remained under the current climatic conditions, while the other half were assigned to climate change simulation plots (hereafter, CCS) in which we manipulated temperature and rainfall (Fig. 1A). Temperature in climate change simulations was manipulated with hexagonal open-top chambers (Marion et al., 1997). Chambers were built with transparent acrylic (3 mm thick, wavelength transmission 280-750 nm), and they are an efficient method to induce warming in remote regions without access to electric power, as was the case here (Hollister and Webber, 2000). Open-top chambers passively increase air temperature inside them by reducing wind speed, and previous studies conducted in oak forest have shown that they can reach temperatures 1.2-2 °C higher than the external environment (Pérez-Ruiz et al., 2018). Rainfall in CCS plots was reduced with rainout shelters that were built with U-shaped channels of transparent polycarbonate (1.5 mm thick, 10 cm wide, 3 m length) supported on metallic frames above the open-top chambers (Yahdjian and Sala, 2002). Five U-shaped channels were equidistantly arranged on each chamber (25 cm above the chamber) and this allowed us to reduce rainfall by 10-18%. Therefore, CCS plots consisted in open-top chambers covered with rainout shelters (Fig. 1B). Control plots, on the other hand, were fenced with wire mesh (13 mm openness) to prevent access of rodents that consume acorns and oak seedlings (Fig. 1C). It was not necessary to fence CCS plots because the walls of open-top chambers prevented the access of herbivores.
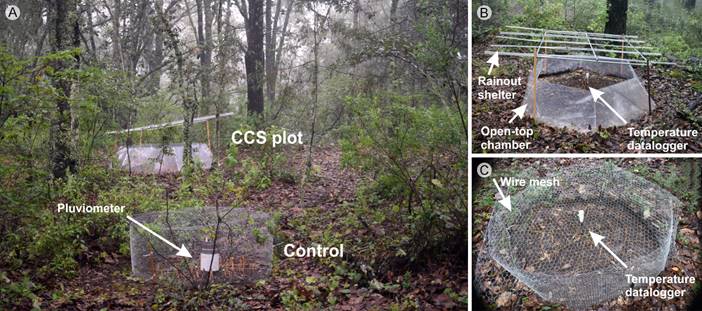
Figure 1: A. experimental plots mounted beneath the canopy of the Quercus ariifolia Trel. stand for this study; B. detail of a control; C. detail of a CCS plot. The devices pointed within experimental plots are the automatized sensors used for monitoring temperature and rainfall. Pictures: E. J. Sánchez-Montes de Oca.
The responses of Q. ariifolia seedlings under current and future climatic conditions were assessed by sowing acorns within the experimental plots. To obtain plant material, we looked for adult trees with acorns across the study site. We identified 30 Q. ariifolia individuals meeting this condition and collected 100 mature acorns beneath their canopies at the beginning of September 2016. These acorns were pooled in a single sample and taken to the laboratory. As acorns collected in the field can be parasitized by insect or fungi, or they may have lost viability due to desiccation (Ramos-Palacios et al., 2014), we used the float test to separate viable and unviable acorns. For this, we removed acorn cupules and nuts were placed in water by 2 h. After that, sunken acorns were assumed as viable and floating acorns were assumed as unviable (Gribko and Jones, 1995). This resulted in 1878 potentially viable acorns (62.6% of viability), which were stored in hermetic plastic bags at 5 °C to prevent germination until their use in the field.
On 16 September 2016, we randomly selected 200 viable acorns and grouped them in 20 batches (10 acorns per batch) that were taken to the field and randomly assigned to ten controls and ten CCS plots. Acorns were sowed in random positions at 2 cm depth to emulate the behavior of field mice (Peromyscus spp.), which are their main dispersers in the region (Ramos-Palacios et al., 2014). Sowing positions were marked with small wood stakes and seedling emergence and survival were monitored every seven days until 27 January 2017 (19 weeks across the rainy season). Seeding emergence was defined by the emergence of epicotyls, while seedling death was assumed when all aerial structures of seedlings (leaves and stems) were fully wilted. At the end of the experiment, ungerminated acorns were recovered and taken to the laboratory to assess their viability with the tetrazolium test. For this, we performed a small incision on pericarps of acorns and incubated them 24 h in 1% solution of 2,3,5-triphenyltetrazolium chloride. If embryos are alive, the colorless tetrazolium salt reacts with the hydrogen released by active dehydrogenase enzymes and it is reduced to formazan, a non-diffusible red dye that stains the embryos (Bewley et al., 2013).
Microclimate monitoring
We used automatized sensors to assess differences in temperature and rainfall between controls and CCS plots (Fig. 1). For this, a datalogger (HOBO U23-Pro-V2, Onset Computer Corporation, Bourne, USA) was established 20 cm above ground at the center of each experimental plot, which was programmed to record temperature every hour during the rainy season. At the end of the experiment, these data were used to compute the average, maximum and minimum air temperature at each experimental plot (n=10 per climate treatment). We also established automatized pluviometers (HOBO S-RGB-M002, Onset Computer Corporation, Bourne, USA) at five randomly selected plots of each climate treatment and programmed them to measure the accumulated rainfall during the experiment (n=5 per climate treatment). Additionally, we weekly measured soil water content in all experimental plots using a time-domain reflectometer (FieldScout TDR 300, Spectrum Technologies, Aurora, USA). These measurements were performed with probes of 7.6 cm because the upper soil layer in the study site, where oak seedlings establish, is shallow (10 cm to the bedrock). Each week, we took six measurements on random points within each experimental plot and these values were automatically averaged by the device. By the end of the experiment, weekly values of soil water content were averaged for each plot (n=10 per climate treatment) to assess whether they differ between climate treatments.
Functional traits of seedlings
On 28 January 2017, after finishing the monitoring of emergence and survival of seedlings, we randomly selected an individual from each experimental plot to assess their functional traits (n=10 per climate treatment). No more seedlings were selected because most measures described below should be performed in less than 1 h across all experimental plots. On each seedling, we marked three healthy leaves (i.e., with no symptoms of insect damage or wilting) with small paper tags and measured chlorophyll fluorescence with a portable fluorometer (MII-PAM II, Heinz Walz GmbH, Effeltrich, Germany). This device was also used to measure the foliar temperature (FT) and the photosynthetic photon flux density (PPFD) in the leaf environment. These measurements were performed at predawn (05:00-06:00 h), midday (12:00-13:00 h) and sunset (17:00-18:00 h). Chlorophyll fluorescence data gathered at predawn was used to estimate the maximum quantum yield of photosystem II, which is only possible in dark-adapted leaves (Maxwell and Johnson, 2000). This variable was computed as the ratio between variable (FV ) and maximum (FM ) fluorescence as: FV /FM = (FM - /F0 )/FM , where F0 is the fluorescence emitted by dark-adapted leaves when they are excited with a low-intensity pulse of actinic light (≈50 μmol photon m-2 s-1) and FM is their maximum fluorescence when a saturating pulse of actinic light is applied (≈2500 μmol photon m-2 s-1). Plants growing under optimal conditions had FV /FM between 0.83 and 0.85, but this ratio decreases as environmental stress increases (Maxwell and Johnson, 2000). Chlorophyll fluorescence measured at midday and sunset was used to estimate the effective quantum yield of photosystem II (ΦPSII), which requires light-exposed leaves. This variable was computed as: ΦPSII = (F'M - F)/FM , where F is the basal fluorescence emitted by leaves under steady-state illumination and F'M is their maximum fluorescence when a saturating pulse of actinic light is superimposed on environmental illumination (≈2500 μmol photon m-2 s-1). As environmental stress reduces the capability of electron transport chains of chloroplasts to accept light-energy harvested by photosystems, this results in increased emission of fluorescence. Hence, values of F approach to those of F'M in plants growing under harsh environmental conditions, resulting in lower ΦPSII values (Maxwell and Johnson, 2000).
Besides emitting fluorescence, plants also dissipate the exceeding light-energy as heat through the xanthophyll cycle. For this reason, we also estimated the non-photochemical quenching (NPQ) of seedling leaves, which refers to reductions in the emission of chlorophyll fluorescence (i.e., quenching) due to the xanthophyll cycle (Huner et al., 2008). This variable was estimated as: NPQ = FM /F'M and their values are expected to increase with increasing environmental stress. Finally, values of ΦPSII and PPFD were used to estimate the electron transport rate (ETR) in the thylakoid membrane of chloroplasts. This variable was computed as: ETR = PPFD × 0.85 × 0.5 × ΦPSII, where 0.85 and 0.5 are constants that account for the fraction of light absorbed by photosystems II and I, respectively (Ritchie and Bunthawin, 2010). Under steady state illumination, ETR decreases with increasing environmental stress because they are directly related with ΦPSII.
At sunset, we assessed chlorophyll content (Chl) of leaves with a portable reflectometer (SPAD 502, Konica Minolta, Japan). We measured this variable because large fractions of plant nutrients (mainly nitrogen) are allocated to the synthesis of chlorophyll molecules (Richardson et al., 2002). Hence, if climate change alters the nutritional status of plants and/or their metabolic pathways of resource allocation, this could affect chlorophyll contents of leaves and alter their photosynthetic performance. Because the device we used provides Chl values in relative units (SPAD units), they were converted in net Chl values (mg cm-2) using the equation proposed by Coste et al. (2010) as: Chl = (117.10 SPAD)/(148.84 - SPAD). For the statistical analyses described below, values of FT, PPFD, F V /F M , ΦPSII NPQ, ETR and Chl were averaged across leaves of the same seedling to avoid pseudoreplication.
On 29 January 2017, a day after performing the former measurements, we harvested the stems, roots and leaves of selected seedlings. These plant organs were rinsed with water to remove dust and dried with softs paper towels to weigh them in situ using an analytical balance (Cubis, Sartorius, Gotinga, Germany) connected to a portable power source. This allowed us to determine the fresh biomass of each plant organ (BFstem , BFroot and BFleaves ). These plant organs were later stored in botanical presses, taken to the laboratory and dried in air-forced stoves (60 °C , 72 h) to obtain their dry biomasses (BFstem , BFroot and BFleaves ). These data were used to compute the percent water content (PWC) of each plant organ as: PWC = (BF - BD)/BF. Values of PWC of different plant organs were latter added to estimate the PWC of each individual seedling, and this variable was used as surrogate of the water status of plants in the field.
Leaves of each seedling were scanned (Scanner Perfection V39, Epson, Suwa, Japan) and images were processed with MideBMP 4. 2 (Ordiales-Plaza, 2000) to determine their foliar areas (LA). These data were used to estimate the specific leaf area (SLA) as: SLA = LA/BD leaves . As plants subjected to thermal and/or water stress usually produce smaller and thicker leaves, SLA decreases with increasing environmental stress (Quero et al., 2006). We also measure the length (SL) and basal width (SW) of seedling stems with a digital caliper (CALDI-6MP, Truper, Mexico City, Mexico) to estimate the net growth rate (NGR) of stems as: NGR = SL/E; here, E is the age of each seedling, estimated as the number of days elapsed since shoot emergence. We also estimated the stem wood density (SWD) of seedlings as: SWD = BDstem /Vstem , where Vstem is the stem volume. As seedling stems are conical, Vstem was computed as: Vstem = (SW/2)2 × π × SL/3. Finally, we estimated the root:shoot ratio of each seedling as BDroot /(BD stem + BDleaves ).
Statistical analysis
Average, maximum and minimum air temperatures recorded with dataloggers, as well as accumulated rainfall and soil water content, were compared between climate treatments (controls vs. CCS) with Student t-tests. Seedling emergence rates were estimated with the Kaplan-Meier’s method and compared between climate treatments with a Gehan-Wilcoxon test (Kleinbaum and Klein, 2005). The same statistical procedures were used to compare seedling survival rates but, in this case, only emerged seedlings were included in the analyses.
Values of FT were compared between climate treatments at the different moments of the day (predawn, midday and sunset) using repeated measures ANOVA and post hoc Tukey tests. As values of FV/FM and ΦPSII are indicative of the quantum yield of photosystem II and they are expected to vary across measuring times, we also used repeated measures ANOVA to compare them. Because values of PPFD, NPQ and ETR were estimated for light-exposed plants, they were compared between climate treatments with repeated measures ANOVA that considered values taken at midday and sunset only. The other functional traits of seedlings (Chl, PWC of stems, roots, leaves and whole seedlings, SLA, NGR, SWD and root:shoot ratios) were compared between climate treatments with Student t-tests. The datasets that support the analyses described in this section are freely available for readers as online resources (see Badano, 2018). The statistical analyses described in this section were performed with R 3.3 (R Core Team, 2016).
Results
Microclimate
Air temperature was significantly increased in CCS plots. Average temperature in these experimental units was 1.6 °C higher than in controls, while maximum and minimum temperatures were 2.4 and 2.0 °C higher than in controls, respectively (Table 1). Furthermore, rainout shelters significantly reduced rainfall in CCS plots, where precipitation was 13.9% lower than in controls (Table 1). These results indicate that climatic conditions in CCS plots approached to those predicted to occur during the rainy season by the middle of this century and support the results described below about the potential effects that climate change could have on oak seedlings. Furthermore, this manipulation of temperature and rainfall significantly reduced soil water content, which was 24.1% lower in CCS plots than in controls (Table 1), and this indicates that changing climate can substantially reduce water availability for recently emerged oak seedlings.
Table 1: Values of air temperature (average, minimum and maximum), rainfall and percent water content of the soil recorded in controls and CCS plots during the oak recruitment season (September 2016-January 2017). Values are averages (±95% I.C.). The last column of the table shows the results of the Student t-tests used to compare values between climate treatments (critical α=0.05).
Climatic variable | Controls | CCS plots | Results of t-tests |
Average air temperature (°C) | 12.75±0.13 | 14.33±0.12 | t=17.32, df=18, p<0.01 |
Maximum air temperature (°C) | 24.47± 0.37 | 26.88 ± 0.36 | t=9.20, df=18, p<0.01 |
Minimum air temperature (°C) | -0.14±0.15 | 1.91±0.13 | t=20.01, df=18, p<0.01 |
Rainfall (mm) | 325.96±1.15 | 280.44±1.14 | t=38.87, df=8, p<0.01 |
Percent water content (%) | 31.94±0.835 | 24.25±0.766 | t=13.29, df=18, p<0.01 |
Seedling emergence and survival
The tetrazolium test performed on ungerminated acorns recovered from experimental plots in January 2017 indicated that none of them was viable (60% of acorns from CCS plots and 57% of acorns form controls did not germinate and were unviable). Hence, we assumed that germination would no longer occur hereafter. No differences in seedling emergence rates were recorded between controls and CCS plots (Gehan-Wilcoxon statistic=1.17, p=0.23). In both climate treatments, seedling emergence increased until day 60, stabilizing around 42% (Fig. 2A). Conversely, seedling survival was significantly higher in controls than in CCS plots (Gehan-Wilcoxon statistic=2.92, p<0.01). After 100 days of experiment, seedling survival stabilized around 77% in controls and around 51% in CCS plots (Fig. 2B).
Functional responses of seedlings
No differences in percent water content of stems, leaves, roots or whole seedlings were found between climate treatments (Table 2). Values of specific leaf area and net growth rate of seedlings were lower in CCS plots than in controls, but the opposite occurred with stem wood density and root:shoot ratios (Table 2). Furthermore, seedlings that grew in CCS plots had significantly higher chlorophyll content than those from controls (Table 2).
Table 2: Functional traits measured on seedlings from controls and CCS plots after finishing the field experiment, including percent water content (PWC) of leaves, stems, roots and whole seedlings, specific leaf area (SLA), net growth rate of stems (NGR), stem wood density (SWD), root:shoot ratio and chlorophyll content (Chl). Values are averages (±95% I.C.). The last column of the table shows the results of the Student t-tests used to compare values between treatments (critical α=0.05).
Functional traits | Controls | CCS plots | Results of t-tests |
PWC of leaves (%) | 41.00±1.63 | 39.53±1.40 | t=1.34, df=18, p=0.20 |
PWC of stems (%) | 50.83±2.10 | 50.94±2.38 | t=0.07, df=18, p=0.95 |
PWC of roots (%) | 33.28±1.78 | 35.52 ±1.93 | t=1.69, df=18, p=0.12 |
PWC of seedlings (%) | 41.94±1.03 | 40.38±1.51 | t=1.67, df=18, p=0.14 |
SLA (mm2/mg) | 14.35±0.29 | 12.00±0.30 | t=10.96, df=18, p<0.01 |
NGR (mm/day) | 0.83±0.04 | 0.44±0.03 | t=15.58, df=18, p<0.01 |
SWD (mg/mm3) | 0.67±0.06 | 1.41±0.06 | t=17.53, df=18, p<0.01 |
root:shoot ratio | 1.29±0.06 | 2.76±0.07 | t=29.99, df=18, p<0.01 |
Chl (mg cm-2) | 24.54±0.24 | 32.71±0.28 | t=43.07, df=18, p<0.01 |
Foliar temperatures of seedlings were higher in CCS plots than in controls (F(1.18)=566.33, p<0.01), but these differences varied among measuring times (F(2.36)=3768.07, p<0.01) and interacted with climate treatments (F(2.36)=6.01, p=0.01). In average, seedling leaves in CCS plots were 2.4 °C warmer than those from controls, but the largest difference in foliar temperature occurred at midday (Fig. 3A). These results indicate that there was an effective transmission of the microenvironmental warming induced with open-top chambers to the seedlings.

Figure 3: Variables (average ± 95% I.C.) measured with the portable fluorometer on leaves of seedlings of Quercus ariifolia Trel. from controls (solid bars) and CCS plots (empty bars). These variables include: A. foliar temperature; B. quantum efficiency of photosystem II (B; for predawn measures, and for midday and sunset measures); C. non-photochemical quenching of chlorophyll fluorescence; D. electron transport rate in the thylakoid membrane of chloroplasts. Different letters above the bars indicate significant differences between values (critical α of Tukey tests=0.05).
Quantum yield of photosystem II (FV /FM at predawn, and ΦPSII at midday and sunset) differed between climate treatments (F(1.18)=196.78, p<0.01) and measuring times (F(2.36)=480.12, p<0.01). Significant effects were also found for the interaction of these factors (F(2.36)=36.73, p<0.01). No differences in maximum quantum yield (FV/FM) were recorded between climate treatments at predawn, but effective quantum yields (ΦPSII) during daytime were higher in CCS plots than in controls (Fig. 3B). Conversely, non-photochemical quenching was higher in controls than in CCS plots (F(1.18)=85.46, p<0.01), but these values also differed between midday and sunset measures (F(1.18)=3110.99, p<0.01) and interacted with climate treatments (F(1.18)=65.488, p<0.01). These analyses indicated that control seedlings had higher non-photochemical quenching at midday only, while no differences between climate treatments occurred at sunset (Fig. 3C).
Photon flux densities measured on seedling leaves were higher at midday than at sunset (F(1.18)=28975.21, p<0.01), but no differences were found between climate treatments (F(1.18)=0.39, p=0.55). This indicates that open-top chambers and rainout shelters did not interfere with the light environment within CCS (photon flux density in controls was 373.73±6.12 μmol m-2-s-1 at midday and 30.37±0.87 μmol m-2-s-1 at sunset; photon flux density in CCS plots was 371.00±5.22 μmol m-2-s-1 at midday and 30.50±0.91 μmol m-2-s-1 at sunset). Hence, these results validate the comparisons of electron transport rates between climate treatments (i.e., differences in photon flux density may bias electron transport rates). Values of electron transport rates differed between controls and CCS plots (F(1.18)=192.52, p<0.01) and between measuring times (F(1.18)=16212.54, p<0.01). Interactive effects between these factors were also found (F(1.18)=212.86, p<0.01). Seedlings from CCS plots had higher electron transport rates than those from controls at midday, but values of this variable were lower at sunset and no differences were found between climate treatments at this time (Fig. 3D).
Discussion
Converse to our expectations, increased temperature and reduced rainfall during the rainy season had no effects on the emergence of Q. ariifolia seedlings in the study site. This suggests that, despite the elevated recalcitrance reported for seeds of most oak species (Zavala-Chávez and García-Moya, 1996; Zavala-Chávez, 2001; Kettle, 2012), changing climatic conditions would not promote desiccation of Q. ariifolia acorns beyond the levels that occur under the current climate and, therefore, their effects on seedling emergence may be negligible. Nevertheless, these results must be taken with caution because seasonally dry forests are subjected to large interannual variation in climatic conditions (Vieira and Scariot, 2006). For instance, the nearest weather station, located in a site without canopy cover at 4 km from our experiment (21°56'21"N, 100°35'02"W, 1898 m elevation; it belongs to a private mining company), recorded 610 mm of rainfall between July 2016 and January 2017. According to the historical climatic records of this region, this rainfall level is indicative of a wet year (Medina-García et al., 2005). Hence, even when only 64% of that rainfall reached the forest understory (see Table 1; 46% of rainfall would be intercepted by the forest canopy), our results suggest that the predicted reductions in precipitation under climate change scenarios would not be large enough to reduce the emergence of Q. ariifolia seedling in wet years. However, these effects could be quite different in dry years, when similar reductions in percent rainfall could lead to conditions of extreme drought that, combined with higher temperatures, may promote the quick desiccation of recently released acorns and impair their germination. In this way, our results are not fully conclusive about the effects that climate change may have on the emergence of Q. ariifolia seedlings, and experiments covering the interannual variation in rainfall are required for determining these effects.
Despite this lack of effects on the emergence of seedlings, our experiment indicated that changing temperature and rainfall can impair the survival of early life-cycle stages of Q. ariifolia, also promoting shifts in their functional traits. As seedlings were protected from herbivory and no symptoms of pathogen infection were detected during the experiment, wilting was the unique cause of mortality. Hence, we infer that the lower survival in CCS plots was due to the enhanced drought that resulted from climate manipulation. In other seasonally dry forests of America, it was reported that mortality of tree seedlings due to desiccation and wilting is usually low during the rainy season, but survival can be reduced by more than 40% during the first dry season they face (McLaren and McDonald, 2003; González-Salvatierra et al., 2013; Badano et al., 2015). The experiment conducted for this study suggests that increased temperature and lower rainfall will cause similar reductions in the survival of Q. ariifolia seedlings but, in our case, it is important to note that these effects took place in the rainy season. Therefore, while our findings indicate that climate change will enhance mortality of the earliest life-cycle stages of Q. ariifolia during the rainy season, it is feasible to propose that changing climatic conditions will also cause dramatic decreases in seedling survival in the succeeding dry season. Such an increased mortality of oak seedlings due to higher temperature and lower rainfall could then impair the recruitment processes that support the prevalence of Q. ariifolia stands in seasonally dry forest of Mexico and this, in turn, may increase the local extinction risks of this endemic oak.
The shifts in functional traits that we observed in seedlings from CCS plots concurred with those of plants developed under drought stress. These seedlings had lower net growth rates and higher stem wood densities than seedlings from controls and this concurs with symptoms of sclerophylly (i.e., presence of thicker and harder leaves that resist loss of moisture), which are linked with shifts in functional traits addressed to promote plant survival in dry environments (Shao et al., 2008). As water is required for the expansion of cells in active meristems (Palacio et al., 2014), lower net growth rates and higher stem wood densities of seedlings from CCS plots could be explained by the lower soil water contents that we recorded in these experimental units. This may also explain why seedlings from CCS plots had smaller specific leaf areas than those from controls, as reductions in leaf areas and increases in leaf thickness contribute to prevent water loss through transpiration and optimize photosynthesis under drought conditions (Quero et al., 2006; Lambers et al., 2008; Guerfel et al., 2009; Ramírez-Valiente et al., 2010). Additionally, the higher root:shoot ratios displayed by seedlings from CCS plots, as compared with control seedlings, suggest that they prioritized the development of roots over that of aerial plant organs. In most plants, these shifts in root:shoot ratios are addressed to optimize water uptake and maintain positive water balances when they are subjected to thermal and/or drought stress (Kozlowski and Pallardy, 2002;Lambers et al., 2008; Yin et al., 2008). Increased root:shoot ratios, together with smaller specific leaf area, may partially explain why seedlings from CCS plots maintained similar percent water contents than those from controls, at least during the rainy season in which our experiment was conducted.
The exposure of plants to elevated temperatures and water shortage conditions may damage their photosystems and this effect is returned in lower quantum yields of photosystem II, as compared with those of plants growing under favorable conditions (Bukhov and Carpentier, 2004). However, this was not the case of recently emerged seedlings of Q. ariifolia that grew in CCS plots because their maximum quantum yields were similar to those of control seedlings. Thermal and water stress can also reduce the flow of photons harvested by chlorophyll molecules across the electron transport chain of chloroplasts and, consequently, this leads to decreases in the overall photosynthetic performance of individuals and promotes the dissipation of excesses of light-energy as fluorescence and/or heat through the xanthophyll cycle (Adams and Demmig-Adams, 2004). In our case, seedlings from CCS plots had higher effective quantum yields, lower non-photochemical quenching, and higher electron transport rates than those from controls, suggesting that simulated climate change promoted their light-handling efficiencies. These results are contradictory with those of studies evaluating the effects of thermal and/or water stress on plants (e.g., Quero et al., 2006; Badano et al., 2018; Pérez-Ruiz et al., 2018) and, as far as we are aware, no previous studies have reported such positive responses in tree seedlings exposed to change conditions. Instead, these results, allow suggesting that some Q. ariifolia seedlings may be able to tolerate warming and drought in the future, and the higher photosynthetic performance that we recorded in individuals from CCS plots may be due to interactive effects that resulted from the shifts in their physiological and morphoanatomical traits. For instance, if smaller specific leaf areas and higher root:shoot ratios contributed to regulate water balances in CCS seedlings and allowed them to maintain similar water contents as control seedlings, this may have prevented the blocking of electron transport chains of chloroplasts. In consequence, their light-handling efficiencies (measured as effective quantum yield, non-photochemical quenching, and electron transport rate) should not be strongly reduced by increased warming and drought. Furthermore, seedlings that survived in CCS plots until the end of the experiment had higher chlorophyll contents than control seedlings, and this may have enhanced their capability for harvesting light. This combination of morphoanatomical (smaller specific leaf area and higher root:shoot ratios) and physiological shifts (higher chlorophyll contents) may explain why seedlings from CCS plots had higher quantum yields and electron transport rates, but lower non-photochemical quenching, that control seedlings. In this way, although experiments conducted under fully controlled conditions are required for disentailing how interactions between morphoanatomical and physiological shifts improve the performance of Q. ariifolia seedlings emerged under intensified warming and drought, our field experiment indicates that these interactions may be important for surviving individuals.
The lower survival recorded in CCS plots, however, did not support our hypothesis stating that these shifts in morphoanatomical and physiological traits would contribute to maintain mortality levels of recently emerged Q. ariifolia seedings close to those observed under the current climate. These results lead to two contrasting views about the ultimate consequences of climate change on this endemic oak from seasonally dry forests of Mexico. On the one hand, the lower survival of seedlings emerged during the rainy season may reduce the replacement rates of dead trees with new individuals, hence increasing the local extinction risk of the species across its current distribution range. This poses a great challenge for conservation biologists because preventing the extinction of tree species that are highly susceptible to climate change will require drastic lines of action, such as their assisted migration to zones that will be climatically suitable for their development in the future (McLachlan et al., 2007). On the other hand, it can be proposed that warmer and drier conditions may act as selective pressures in the future, which will allow the survival of individuals (seedlings) displaying trait shifts matching with these climates, while they will eliminate individuals lacking these putative adaptations. Although we were unable to determine whether dead seedlings from CCS plots displayed shifts in morphoanatomical and physiological traits, their lower survival and their marked differences in functional traits, as compared to control seedlings, support this latter proposal. Hence, if changing climate constitutes a selective pressure, this may produce changes in the genetic composition of tree species in the future and cause evolutionary processes. This latter suggestion is supported by some studies conducted with American oaks, which proposed that the wide variety of functional traits within species constitute pre-adaptations (i.e., traits that fulfill a function under particular environmental conditions, but that can fulfill new functions under other conditions) that may allow oaks to occupy novel environmental spaces, which in turn enhances their diversification potential and confers them some degree of tolerance to changing climatic conditions (Cavender-Bares et al., 2004; 2011). Nevertheless, determining which of these two scenarios is more likely to occur (i.e., extinction or adaption) requires more detailed experiments which, besides assessing the functional traits of seedlings, must also assess genetic features.