1. Introduction
Two thirds of the Earth’s surface is covered by oceans, providing a well-known source of aerosol particles that have the potential to nucleate cloud droplets and ice crystals, influencing cloud albedo and precipitation, and hence, climate. Although marine aerosol particles are ubiquitous in the atmosphere, their physical and chemical properties are poorly understood (Bigg and Leck, 2001; Heintzenberg et al., 2004; Gantt and Meskhidze, 2013). The major components of primary marine aerosol are inorganic sea salt and organic matter. The latter can be cellular (e.g., bacteria, phytoplankton, and diatoms) and extracellular (e.g., exopolymeric substances; EPS) (Yoon et al., 2007; Vignati et al., 2010). While organic matter is highly concentrated in the sea surface microlayer (SML), the sea salt content is rather constant for a few meters below the surface. Sea salt particles are injected into the atmosphere together with marine organic matter by sea spray as a result of bubble bursting and wave activity (Facchini et al., 2008; Gantt and Meskhidze, 2013). Vignati et al. (2010) estimated the global sea salt emissions to be 24 Tg year-1, whereas the sub-micron organic matter emissions from sea spray were found to be 8.2 Tg year-1.
Marine aerosol particles can influence mixed-phase and cirrus cloud formation because a fraction of them are able to act as ice nucleating particles (INPs), facilitating ice crystal formation via different heterogeneous ice nucleation pathways (e.g., Bigg, 1973; Schnell and Vali, 1975; Schnell, 1975, 1977, 1982; Rosinski et al., 1987, 1988; Mason et al., 2015; DeMott et al., 2016; McCluskey et al., 2017, 2018; Welti et al., 2018; Creamean et al., 2018; Si et al., 2018; Ladino et al., 2019; Gong et al., 2020). As summarized by Burrows et al. (2013), marine microorganisms, EPS aggregates, glassy organics, and crystalline hydrated NaCl are types of aerosol particles that have the potential to nucleate ice in marine environments. However, whether the marine INPs are dominated by a specific aerosol type, and under what conditions, remains unclear due to the limited number of field and laboratory studies (Kanji et al., 2017).
Recent studies have addressed some of the aforementioned gaps in knowledge. For example, laboratory studies have shown that crystalline salts (e.g., Instant Ocean and NaCl), organic matter (e.g., amorphous sucrose), and a variety of marine microorganisms (e.g., Nanochloris atomus, Emiliania huxleyi, Vibrio harveyi, and Prochlorococcus) can efficiently nucleate ice via deposition nucleation at temperatures below -40 ºC (e.g., Wise et al., 2012; Wagner and Mohler, 2013; Schill and Tolbert, 2014; Ladino et al., 2016; Wolf et al., 2019). At warmer temperatures, Knopf et al. (2011) and Wilson et al. (2015) found that the planktonic diatom species Thalassiosira pseudonana (and their exudates) was able to efficiently nucleate ice via immersion freezing, with freezing temperatures as high as -23 ºC. In mesocosm studies, Wang et al. (2015) and McCluskey et al. (2017) found that the concentration of INPs was significantly enhanced during a phytoplankton bloom. The authors suggest that higher INP concentrations are linked to the presence of heterotrophic bacteria and organic species in the sea spray aerosol. More recently, Tesson and Šantl-Temkiv (2018) found that the marine aquatic microalgae Polarella glacialis was able to nucleate ice via immersion freezing at temperatures close to -6 ºC. As shown by Creamean et al. (2019), biological INPs in the Arctic can be emitted into the atmosphere by marine waters as a result of phytoplankton blooms during summer. In both Arctic (Wilson et al., 2015; Irish et al., 2017) and tropical (Gong et al., 2020) waters, INP concentrations have been observed to be either enriched and depleted in the SML in comparison to bulk surface water (BSW) samples, likely dependent on complex interactions between biological, oceanographic, and meteorological conditions.
Burrows et al. (2013) and Yun and Penner (2013) used global climate models to investigate the potential impacts of marine aerosol on the Earth’s radiative balance and the hydrological cycle. Both studies predicted a significant influence of marine organic aerosol (MOA) on cloud properties in the Southern Ocean, remote from terrestrial aerosol sources. In remote areas, MOA could also contribute to the INP concentration significantly where the presence of mineral dust is limited, as is the case for continental biological aerosol (Pratt et al., 2009; Prenni et al., 2009; Pöschl et al., 2010). Recent studies by Wilson et al. (2015), Vergara-Temprado et al. (2017), and McCluskey et al. (2019) reached similar conclusions.
Burrows et al. (2013)) and Yun and Penner (2013) mentioned the urgent need to conduct field studies in marine environments as well as laboratory studies, using marine aerosol particles to reduce uncertainties in global climate predictions. Most notably, there is a limited number of experimental studies focusing on INPs from airborne particles and SML waters in tropical latitudes. Therefore, the currently available parametrizations to model marine INPs may underestimate the role that marine tropical oceans play in the global distribution of INPs.
With the aim to contribute to the understanding of the ice nucleating properties of the marine aerosol in tropical latitudes, a device to quantify the ice nucleating abilities from different types of aerosol samples has been constructed by the Micro and Mesoscale Interactions Group at the Instituto de Ciencias de la Atmósfera y Cambio Climático of the Universidad Nacional Autónoma de México (UNAM). Samples collected from the SML and BSW in the Gulf of Mexico (GoM) and Saanich Inlet (Vancouver Island, Canada), were analyzed using the new device.
2. Methods
2.1 Sampling locations
Two different sampling locations were chosen for this study: (i) Saanich Inlet, off Vancouver Island (VI), Canada; and (ii) the southern GoM. Sampling at VI took place in Patricia Bay (48º 39’ N, 123º 28’ W) as shown in Figure 1a. SML samples (ca. 25 mL) were collected on two different days (March 21 and 22, 2018) and at three different stations using a glass plate (section 2.2). The sampling locations (A, B, and C in Fig. 1a) were approximately 1 km apart, with location A about 1 km from the dock at the Institute of Ocean Sciences. The samples were collected in triplicate and kept frozen (ca. -4 ºC) while transported from Canada to Mexico.

Fig. 1 Sampling sites: (a) at Saanich Inlet, Vancouver Island (VI), and (b) Dzilam de Bravo, Gulf of Mexico (GoM) (Google Earth, 2019). At both locations, individual stations were separated by approximately 1 km.
Sampling in the GoM took place at Dzilam de Bravo (21º 23’ N, 88º 52’ W) on the Yucatan Peninsula (Fig. 1b) as part of the African Dust and Biomass Burning Over Yucatan (ADABBOY) project. Dzilam de Bravo is 107 km away from Merida (the capital and most populated city in the Yucatan state) and 79 km from Progreso (one of the largest harbors in the state). Samples from the SML and BSW were collected (in triplicate) on April 17, 2018 at 10 different stations, separated by 1 km as shown in Figure 1b. A cold front affected the region on April 17, and therefore wave activity was significant in comparison to previous days and also in comparison to conditions in the protected bay off VI. The samples were kept frozen (ca. -4 ºC) while transported to Mexico City.
A total of 48 SML and 10 BSW samples were collected as summarized in Table I. At each sampling station, both at VI and in the GoM, the sea surface temperature (SST), and the salinity were measured with a YSI 85 multiparameter probe.
2.2 Sampling methods
A glass plate, an old and simple but very useful tool to collect SML samples, was used in this study (Harvey and Burzell, 1972; Cunliffe and Wurl, 2014). We used a 30 × 20 cm plate of tempered glass with a thickness of 4 mm (Fig. 2a). To collect a sample from the SML, the glass plate (previously rinsed at least three times with deionized water on both sides) was vertically immersed into the sea surface and then lifted slowly at a constant rate (Cunliffe and Wurl, 2014). As the glass plate was lifted, the SML adhered to it, so it was removed from the glass plate with the help of a neoprene squeegee as shown in Figure 2b. The SML samples were collected in high-density polyethylene (HDPE) amber bottles and stored at -20 ºC prior to their analysis. Note that the temperature of the samples was -4 ºC during their transport to Mexico City, where the DFA analysis were performed. The thickness of the collected SML (h in mm) can be calculated following Eq. (1) from Cunliffe and Wurl (2014):

Fig. 2 (a) Glass plate used in the present study, (b) sea surface microlayer (SML) sample collection procedure, and (c) bulk surface water (BSW) sample collection procedure.
where V is the sample volume in cm3, A the total area of the immersed glass plate in cm2 (i.e., the area of both sides), and N the number of dips per sample (dimensionless). BSW samples were only collected in the GoM (Fig. 2c) at a depth of approximately 1 m using a Niskin bottle (Seabird Coastal).
2.3 The UNAM-droplet freezing assay (UNAM-DFA)
The droplet freezing assay (DFA) has been widely used to study the ice nucleating abilities of different aerosol types (Vali and Stansbury, 1966; Vali, 1971; Lindow, 1983; Conen et al., 2011; Attard et al., 2012; Wright and Petters, 2013; Stopelli et al., 2014; Hill et al., 2014; Budke and Koop, 2015; Whale et al., 2015; David et al., 2019). This method specifically studies the immersion freezing mode, which has been recognized as the most important pathway to form ice crystals in mixed-phase clouds (Murray et al., 2012). Ice formation via immersion freezing occurs when a liquid droplet with an aerosol immersed is exposed to decreasing temperatures. As a consequence of the lower temperature, an ice germ forms at the surface of the aerosol particle causing the droplet to freeze (Murray et al., 2012; Kanji et al., 2017). The UNAM-DFA (Fig. 3) is based on the design by David et al. (2019) and consists of: (i) a thermostat (LAUDA PRO-RP 1090) filled with polydimethylsiloxane as recirculating-cooling liquid; (ii) an aluminum sample holder to support the V-bottom Enzyme-Linked Immunosorbent Assay (ELISA) plate (Corning 3896); (iii) a light-emitting diode (LED) system, and (iv) a video camera (ATVIO, HD 1080P, WDV800SA) to record the freezing experiments.

Fig. 3 Diagram showing the main components of the UNAM-droplet freezing assay (UNAM-DFA). (a) Sample preparation; (b) sample holder, Enzyme-Linked Immunosorbent Assay (ELISA) plate, video camera arrangement, and (c) cross section view of the full setup.
Using an eight-tip micropipette (EPPENDORF 300), 50 µL of sample (i.e., SML or BSW) were transferred into each of the 96 wells of a sterile ELISA plate (Fig. 3a). The plate was then covered with a transparent film to seal the plate and to avoid any interaction between the sample and the ambient laboratory air. The 50 µL in each well corresponds to the volume of a liquid drop with a diameter of ca. 4.4 mm. The loaded ELISA plate was placed into the cooling bath where the temperature decreased from 0 to -40 ºC at a cooling rate of 2.66 ºC min-1 (Fig. 3c). While the temperature decreased, the freezing of each well was monitored and recorded with the video camera. The freezing of each well was determined by the change in its opacity while transitioning from liquid to solid (Fig. 4). The temperature was assumed to be the same in all of the 96 wells at a given time during the temperature ramp and was recorded from the thermostat with an uncertainty of ± 0.01 ºC. Given that the volume of the polydimethylsiloxane decreases as a function of temperature due to thermal contraction, the depth of the ELISA plates was manually controlled with the help of adjustable screws on either side of the metallic support (Fig. 3b).

Fig. 4 Example of an Enzyme-Linked Immunosorbent Assay (ELISA) plate with liquid (red circles) and frozen (yellow circles) wells differentiated according to their appearance.
A video and a file of the temperature as a function of time were obtained from each experiment. The frozen fraction (f) at 1 ºC intervals was obtained combining both information sources following Eq. (2):
where N f is the number of frozen droplets at a specific temperature and N the total droplets of the ELISA plate (i.e., 96). The cumulative INP concentration (L-1) is derived following Eq. (3) (Yadav et al., 2019):
where F uf is the fraction of unfrozen droplets (dimensionless) at temperature T (ºC), and V drop is the drop volume (L).
3. Results and discussion
3.1 UNAM-DFA performance
We performed homogeneous freezing experiments with MilliQ water (18.2 MΩ cm) to assess the performance and limits of the UNAM-DFA. As shown in Figure 5a, the homogeneous freezing curve obtained with the UNAM-DFA is close to the one reported by Tobo (2016). However, the liquid droplets from the present results were found to freeze at lower temperatures than those found in previous studies (e.g., Whale et al., 2015; Irish et al., 2017; Yadav et al., 2019). The variability between the homogeneous freezing curves shown in Figure 5a is not surprising given that the spontaneous freezing of liquid droplets is influenced by its size, the cooling rate, and the details of the method used (i.e., suspended droplets vs. droplets placed on cold stages), among other factors. The homogeneous freezing curve serves as a zero. Any freezing event at higher temperatures is attributed to the presence of impurities, such as aerosol ice nucleating particles (INPs), immersed in the droplets and categorized as heterogeneous freezing.

Fig. 5 (a) Homogeneous freezing activation curves, and (b) ice nucleating particles (INP) concentration calculated for Arizona Test Dust (ATD) samples. The error bars are the representative average variability associated to the homogeneous freezing experiments.
Arizona Test Dust (ATD) was utilized in a secondary experiment to further assess the performance of the UNAM-DFA. ATD can be considered a proxy for natural mineral dust and its ice nucleating abilities in different ice nucleation modes are well known (Kanji et al., 2008; Kanji and Abbatt, 2010; Welti et al., 2009; DeMott et al., 2011; Niemand et al., 2012; Hader et al., 2014; Steinke et al., 2015; Yadav et al., 2019). An aqueous solution of 0.1% w/w ATD (ISO 12103-1; Powder Technology, Inc; A1 ultrafine) in MilliQ water was prepared and analyzed in the UNAM-DFA. Figure 5b shows the relationship between INP concentration (in the form of ATD particles) and freezing temperature. Although the INP concentrations in our samples were lower than those observed by Yadav et al. (2019), the trend with freezing temperature in both data sets is consistent. The small difference found in the concentration range where these two studies overlap can be attributed to the grain size of the ATD, i.e., ultrafine (A1) in the present study versus coarse (A4) used by Yadav et al. (2019).
3.2 Ice nucleating properties of the SML: Gulf of Mexico vs. Vancouver Island
Figure 6 shows an intercomparison of the frozen fraction of the SML samples collected off VI and in the GoM within 3 km of shore. The two sets of samples are clearly distinguishable, with the GoM samples closer to the homogeneous freezing line. Therefore, the SML samples from VI were more efficient at nucleating ice. Note that the variability between stations in the ice nucleating abilities of the SML samples from the GoM is greater than those from VI. While the activation curves of the VI samples range from -9.5 to -18.5 ºC, the GoM samples vary from -21.5 to -35.5 ºC. This is consistent with the VI stations being confined to a relatively enclosed inlet, whereas the GoM stations extended more directly away from the coast into open water.
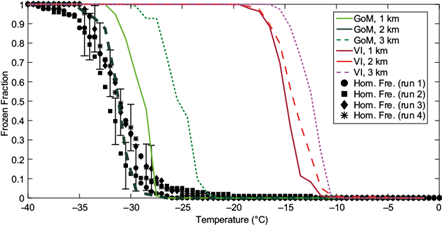
Fig. 6 Frozen fraction curves for the sea surface microlayer (SML) samples collected in the Gulf of Mexico (greenish lines) and off Vancouver Island (reddish lines). The black symbols denote the homogeneous freezing curves, and the error bars are the representative average variability (Fig. 5).
Another way to quantify the ice nucleating ability of a given sample is by the temperature at which 50% of the droplets freeze, denoted as T50. Figure 7a shows that the median T50 values for the VI and GoM SML samples were -13.5 and -28.7 ºC, respectively. Therefore, both the activation scans and the T50 clearly show that the SML samples from VI were significantly more efficient at nucleating ice than those collected in the GoM.

Fig. 7 Box plots for (a) T50 values of the Gulf of Mexico (GoM) and Vancouver Island (VI) sea surface microlayer (SML) samples within 3 km of shore, and (b) the sea surface temperature (SST) measured in the GoM and off VI. Ten GoM and six VI observations were used to build each panel. The top and bottom limits of each box are the 25th and 75th percentiles of the samples, respectively. The median of the samples is represented by the line in the middle of each box. The top and bottom whiskers on each box indicate the maximum and minimum values, respectively.
Given that the same person collected the samples and that the instrumentation used to collect the samples and to analyze them were identical, the differences observed in the results are likely related to the different characteristics of the composition of the SML in the GoM and the mid-latitude Saanich Inlet. Table II shows that the average salinity and sea surface temperature (SST) measured in the GoM are significantly higher than those measured at VI. The lower salinity in the waters off VI is a direct result of the high volumes of river waters delivered to the ocean in that area. Irish et al. (2019) also found higher INP concentrations associated with riverine waters in the Arctic Ocean. While crystalline sea salt particles are very inefficient INPs, via immersion freezing, due to their high solubility (e.g., Kanji et al., 2017), organic aerosol particles of marine origin have been shown to be efficient INPs (e.g., Schnell, 1975; Wilson et al., 2015; DeMott et al., 2016; Irish et al., 2017; Creamean et al., 2018; Gong et al., 2020). Van de Poll et al. (2013) showed how lower SST values positively correlate with the availability of nutrients required for marine production. They found that lower SST values are linked with higher chlorophyll-a. Figure 7 shows that the T50 values in our samples were inversely related to the SST measured at each sampling site.
Table II Summary of the average physicochemical characteristics of the samples collected* in the Gulf of Mexico (GoM) and off Vancouver Island (VI).
Variable | GoM | SD | Number of measurements | VI | SD | Number of measurements |
Sea Surface temperature (ºC) | 25.88 | 0.03 | 10 | 8.74 | 0.05 | 6 |
Salinity (psu) | 34.25 | 7.39 | 10 | 27.94 | 0.13 | 6 |
SD; standard deviation.
*Samples from both sites were collected during the local morning.
3.3 Ice nucleating capacity of the Gulf of Mexico samples: SML vs. BSW
Given that SML and BSW samples were collected at each of the 10 sampling stations in the GoM, it was possible to conduct a direct comparison of the ice nucleating abilities of these two types of samples. Figure 8a shows that the BSW samples were more efficient at nucleating ice as their freezing temperatures are closer to 0 ºC. This is also shown by the T50 values, where the BSW samples had higher T50 values than the SML samples by 4.2 ºC (Fig. 8b).

Fig. 8 a) Frozen fraction curves for the sea surface microlayer (SML; red lines) and bulk surface water (BSW; blue lines) samples collected at the Gulf of Mexico, and (b) box plot of the T50 from the SML and BSW. The error bars in (a) are the representative average variability associated to the homogeneous freezing experiments. On panel (b), the tops and bottoms of each box are the 25th and 75th percentiles of the samples, respectively. The median of the samples is represented by the line in the middle of each box. The top and bottom whiskers on each box indicate the maximum and minimum values, respectively. The red cross indicates an outlier value.
Although the present results are in agreement with those reported by Irish et al. (2017) from SML and BSW samples collected in the Arctic, Wilson et al. (2015), Chance et al. (2018), Irish et al. (2019), and Zeppenfeld et al. (2019) found higher ice nucleation efficiencies in the SML in comparison to deeper waters. More recently, Gong et al. (2020) reported the absence of a clear trend between the INP concentrations in the SML and BSW samples collected off Cape Verde, in the Atlantic Ocean (at 16-24º N). Organic matter and hence INPs can be concentrated in the SML. As mentioned above, during the sampling in the GoM a cold front affected the region. This caused the generation of medium to strong waves, which can enhance organic matter enrichment in the SML (Cunliffe et al., 2013). Additionally, as highlighted by Irish et al. (2017) and Wurl and Obbard (2004), the SML sampling method can play an important role in the thickness of the collected SML and therefore in its observed physicochemical properties. Note that in Irish et al. (2017) and in the present study, a glass plate was used to collect the SML. On the other hand, Wilson et al. (2015), Chance et al. (2018), and Irish et al. (2019) used a rotating drum. Overall, there is a hint that the sampling method and hence the thickness of the sampled SML can impact the measured ice nucleating activity. This potential bias deserves further study.
3.4 Small-scale spatial variability in ice nucleating activity
The ice nucleating activity of the SML and BSW samples collected in the GoM was evaluated as a function of the distance from shore, as shown in Figure 9. The minimum T50 was observed 2 km from shore for both the SML and SBW samples. Beyond 3 km, the T50 values for the BSW were relatively constant (-21.2 ± 0.7 ºC); however, the SML samples showed a higher variability (-25.2 ± 1.7 ºC). Therefore, although a small effect of the coast on the ice nucleating abilities of the SML and BSW waters was observed, this seems to be very local and likely caused by different anthropogenic activities at the coast (e.g., the presence of boats). Although the T50 values from the BSW samples and the distance from the shore did not correlate (r = 0.25, p = 0.48), the T50 values from the SML sample were found to moderately correlate with the distance from the shore (r = 0.52); however, the correlation is not statistically significant (p = 0.11). It was found that the T50 values of the SML and BSW, as a function of the distance from the shore, have a good correlation (r = 074, p < 0.05). This indicates that the properties of the SML and BSW from the GoM may be driven by similar processes such as water mass characteristics (i.e., freshwater fractions) and ecosystem structure.

Fig. 9 T50 values as a function of the distance from shore for the sea surface microlayer (SML) and bulk surface water (BSW) samples collected in the Gulf of Mexico. The error bars depict the representative average variability.
Although the small-scale (i.e., 10 km) spatial distribution of the INPs around Dzilam de Bravo is not constant, the observed variability is not very large. As shown by Wilson et al. (2015) and Irish et al. (2017), significant changes can be found when samples are collected over large distances, much larger than 10 km.
3.5 INP concentrations
Using the frozen fractions shown in Figures 6 and 8a and following Eq. (3), the INP concentrations for the entire data set were calculated and are shown in Figure 10. Although the INP concentrations of the VI and the GoM samples are on the same order of magnitude, the INP activities in the VI samples were found at much higher temperatures than those of the GoM samples. The INP concentrations in the VI SML samples varied from 2.1 × 102 to 9.1 × 104 L-1 water at temperatures ranging from -10.5 to -18.5 ºC. Likewise, the SML and BSW samples from the GoM contained INPs in concentrations from 6.0 × 101 to 1.1 × 105 L-1 water at temperatures below -16.5 ºC. Although the INP concentrations from the VI samples are in agreement with those reported by Wilson et al. (2015) and Irish et al. (2017), the INP concentrations from the GoM samples are two or three orders of magnitude lower, possibly because of the lower overall biological productivity of tropical versus polar and subpolar waters.
4. Conclusions
A droplet freezing assay device, denoted as UNAM-DFA, was built at the Universidad Nacional Autónoma de México to study mixed-phase cloud formation via the immersion freezing mode. The results obtained with the UNAM-DFA are in agreement with those reported by similar DFAs built elsewhere. Given the current lack of data on the ice nucleating abilities of aerosol particles emitted in tropical latitudes, the UNAM-DFA will be very useful to fill the gaps in the current knowledge of how the oceanic emissions may impact atmospheric chemistry and cloud formation.
Samples from the sea-surface microlayer of the GoM were found to be less efficient (lower T0 and T50 values by 12 ºC and 15 ºC, respectively) at nucleating ice than comparable samples collected off VI, on the west coast of Canada. This is likely related to the physicochemical characteristics, including freshwater influences and overall biological productivity, of the GoM and VI waters. Given that neither the GoM nor the VI samples were collected under the influence of coincident phytoplankton blooms, it would be interesting to collect samples during active blooms in the GoM (i.e., October-November) to assess whether the ice nucleating abilities of the SML are higher under such conditions than those observed here.
The bulk surface waters from the GoM were found to have higher ice nucleating activities than the corresponding SML samples, as the T50 values in the bulk waters were 4.2 ºC higher. Although this can be considered somewhat unusual, similar results have also been found in the high Arctic (Irish et al., 2017). The conditions and processes that lead to enrichment of ice-nucleating activity in the sea-surface microlayer deserve further investigation.
For the most part, there was not a clear pattern in the spatial distribution of ice nucleating activity of the SML and BSW samples either in the GoM or off VI. However, more information is required to assess possible causes of the minimum T50 values found 2 km from shore in the GoM. The good correlation between the SML T50 and BSW T50 as a function of the distance from the shore suggests that the properties of both types of samples may be driven by similar processes such as water mass characteristics (i.e., freshwater fractions) and ecosystem structure. It would be interesting to collect SML and BSW samples out in the open Pacific Ocean and further into the central GoM to evaluate whether their ice nucleating abilities differ significantly from those found closer to shore.
Finally, the INP concentrations in the GoM (6.0 × 101 to 1.1 × 105 L-1 water) and VI (2.1 × 102 to 9.1 × 104 L-1 water) samples agreed well with literature data at different locations, with the exception of the SML samples from the GoM, which were substantially lower than other observations. The high INP concentrations found at high temperatures in the VI samples suggest that these waters have the potential to significantly affect mixed-phase cloud formation on a local scale.