1 Introduction
Understanding the internal structure and reactions of nuclear systems from first principles was, for a long time, an important goal of nuclear and particle physics. In this regard, few- and many-body systems provide a unique laboratory for studying nuclear interactions. Recent years have witnessed interesting developments in coherent and incoherent pion photoproduction on the deuteron and light nuclei, since relevant experimental studies have been performed (see Refs. [1-14] and references therein). These reactions can be utilized to investigate the internal structure of hadrons in the non-perturbative domain of Quantum Chromodynamics (QCD) and study the behavior of nucleon resonances in the nuclear medium.
In particular, the reaction
So far, many studies have been carried out dealing with the reaction
In addition, theoretical models which consider polarization observables for the reaction
Therefore, the main aim of the present work is to discuss theoretical uncertainties in the analyses of
Most recently, the sensitivity of the results for single-spin asymmetries and the helicity E-asymmetry in the
Therefore, the present work was motivated to present results for the unpolarized differential and total cross sections as well as for all possible beam, target, and beam-target spin asymmetries of the differential and total cross sections in the photon energy region from near π-threshold to 170 MeV. As elementary amplitudes, the one provided by the unitary isobar MAID-2007 model from [46] and those obtained using the Dubna-Mainz-Taipei dynamical model (DMT-2001) [47] and the chiral MAID model (χMAID-2013) [48] are used. Furthermore, we compare our results for the unpolarized differential cross section with the experimental data from TAPS [4]. In this comparison with experiment we concentrate our discussion on the unpolarized differential cross section, because data for polarization observables are still not available. The calculations presented in this paper are of particular interest for the evaluation of the systematic uncertainties caused by the use of different elementary operators in the analyses of
This paper is structured as follows. In Sec. 2, we briefly outline the theoretical framework used to describe the
2 Theoretical framework
In this section we briefly describe the theoretical framework for the coherent π0-photoproduction reaction on the deuteron. We would like to mention that the general formalism for the
2.1 Cross section and observables
We consider the reaction
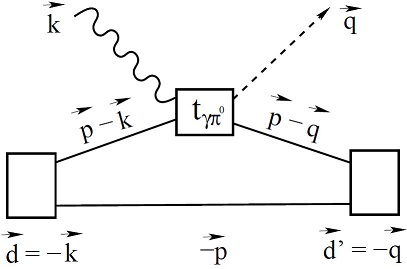
Figure 1 Diagrammatic representation of the
The cross section for arbitrary polarized photons and initial deuterons can be calculated for a given transition
where
where the reduced t-matrix elements are defined by separating the
The kinematic factor
where the deuteron energies in the initial and final states are given by
The invariant energy of the γd system is given as:
Where mπ is the neutral-pion mass.
Following the rules of Ref. [52], we write the differential cross section in terms of the unpolarized differential cross section dσ0/dΩ and the various spin asymmetries Σ,
where
In order to express the unpolarized differential cross section and various polarization observables in terms of the t-matrix, it is appropriate to define the two quantities [50]:
where the convention of Edmonds [53] is used for the Wigner 3j-symbol. The explicit formal expressions for unpolarized differential cross section and spin asymmetries can be derived as follows [28]:
(i) The unpolarized differential cross section:
(ii) The linear photon asymmetry:
(iii) The vector target asymmetry:
(iv) The tensor target asymmetries:
with
(v) The spin asymmetries for circularly polarized photons and vector polarized deuterons:
with
(vi) The spin asymmetries for circularly polarized photons and tensor polarized deuterons:
with
Because the quantity
(vii) The spin asymmetries for linearly polarized photons and vector polarized deuterons:
with
(viii) The spin asymmetries for linearly polarized photons and tensor polarized deuterons:
with
We would like to mention that for θ = 0 and π the spin asymmetries
The deuteron spin asymmetry with respect to circularly polarized photons and the deuteron spin oriented parallel (P) and antiparallel (A) to the photon spin is related to the spin asymmetry
As mentioned in the Introduction, the asymmetry
The general form of the total cross section with inclusion of photon and deuteron polarization effects is obtained from Eq. (7) by integrating dσ/dΩ over the pion spherical angle dΩ and reads [54]:
where the unpolarized total cross section σ0 and the corresponding spin asymmetries
2.2 The
Next, the transition matrix elements
The production operator
The upper index refers to the nucleon on which the elementary operator acts. This means that
where
For the intrinsic part of the deuteron wave function we use the ansatz:
The last two terms
For the elementary
To study the uncertainties caused by the use of different elementary pion photoproduction
operators in the analyses of
3 Results and discussions
In this section, we explore the sensitivity of the results for unpolarized cross sections as well as all various beam, target, and beam-target spin asymmetries of the
3.1 Differential and total cross sections
First, we show in Fig. 2 the energy and angular dependences of the results for unpolarized differential cross section, dσ0/dΩ, using different elementary
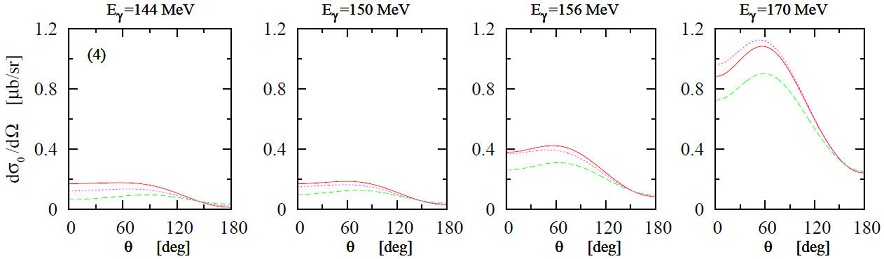
Figure 2 (Color online) The differential cross section for the reaction
Figure 3 shows the results for the
unpolarized total cross section, σ0, for the reaction

Figure 3 (Color online) The unpolarized total cross section for the reaction
3.2 Single-spin asymmetries
Now, we focus our attention on the single-spin asymmetries of polarized photons (Σ) or
polarized deuterons (T11,
T20,
T21 and T22) for
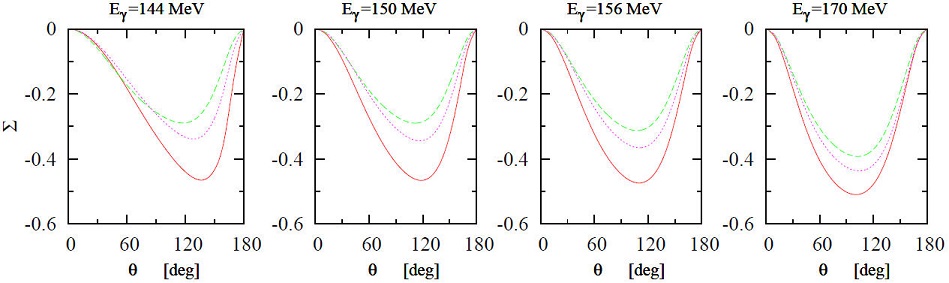
Figure 4 (Color online) Same as in Fig. 2 but for the photon Σ-asymmetry with linearly polarized photons and unpolarized deuterons.
We found that the asymmetry Σ is sensitive to the elementary amplitude, especially in the peak region where sizeable differences are obtained in the pion angle range from 60o to 150o. It has a minimal value around θ ≃ 135o in the case of χMAID-2013 (solid curve) and it is shifted towards lower pion angles in the case of DMT-2001 (dotted curve) and MAID-2007 (dashed curve). It is also very obvious that the computations with different elementary amplitudes are quite different with, in absolute size, a larger Σ-asymmetry predicted using χMAID than the ones obtained with DMT and MAID models. This discrepancy shows up the differences among elementary pion photoproduction operators and means that Σ is very sensitive to the choice of the elementary amplitude, in particular in the vicinity of the peak.
For polarization observables with polarized deuteron targets and unpolarized photon beams, we
present in Fig. 5 the sensitivity of the
results for the vector T11 and tensor
T2M (M = 0,1,2) deuteron spin
asymmetries for the reaction
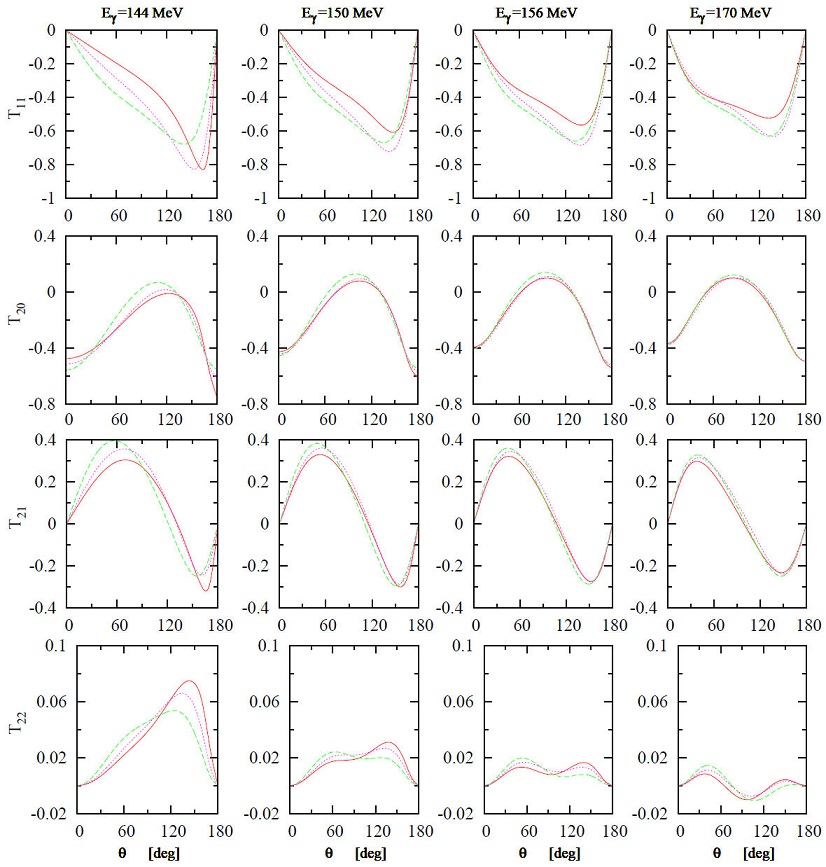
Figure 5 (Color online) Same as in Fig. 2 but for the deuteron asymmetries with vector T11 and tensor T2M (M = 0, 1, 2) polarized deuterons and unpolarized photons.
The results for tensor deuteron spin asymmetries T2M (M = 0,1,2) are also shown in Fig. 4. In general, we see that the results for these asymmetries at the lowest photon energy, Eγ = 144 MeV, are rather different than the results at higher photon energies, especially in the case of T22 asymmetry. The results for T20 and T21 asymmetries using various elementary amplitudes exhibit qualitatively, but not quantitatively, similar behaviors. One can see that the results using different elementary operators are quantitatively rather different, which means that these spin asymmetries are slightly sensitive to the choice of the elementary amplitude. When the photon energy increases, we see that the curves represent the results of T20 and T21 using various elementary amplitudes are close to each other and thus the influence of elementary operators on T20 is small in this case. The T22 asymmetry indicates at photon energies greater than 144 MeV that it has an oscillatory shape. In contrast to the T20 and T21 cases, we find that the results for T22 asymmetry using different elementary amplitudes exhibit qualitatively and quantitatively different behaviors and show up the sensitivity of its results to the elementary amplitude.
A serial system of four columns packed with Eichhornia crassipes and 16 cm radio and 150 cm length is capable of keeping an outlet concentration below 10 ppb
3.3 Beam-target double-spin asymmetries
Here, we report the numerical results for the beam-target double spin asymmetries for

Figure 6 (Color online) Same as in Fig. 2 but for the beam-target double-spin asymmetries with circularly polarized photons and vector polarized deuterons.
Figure 7 shows the results for the
beam-target double spin asymmetries
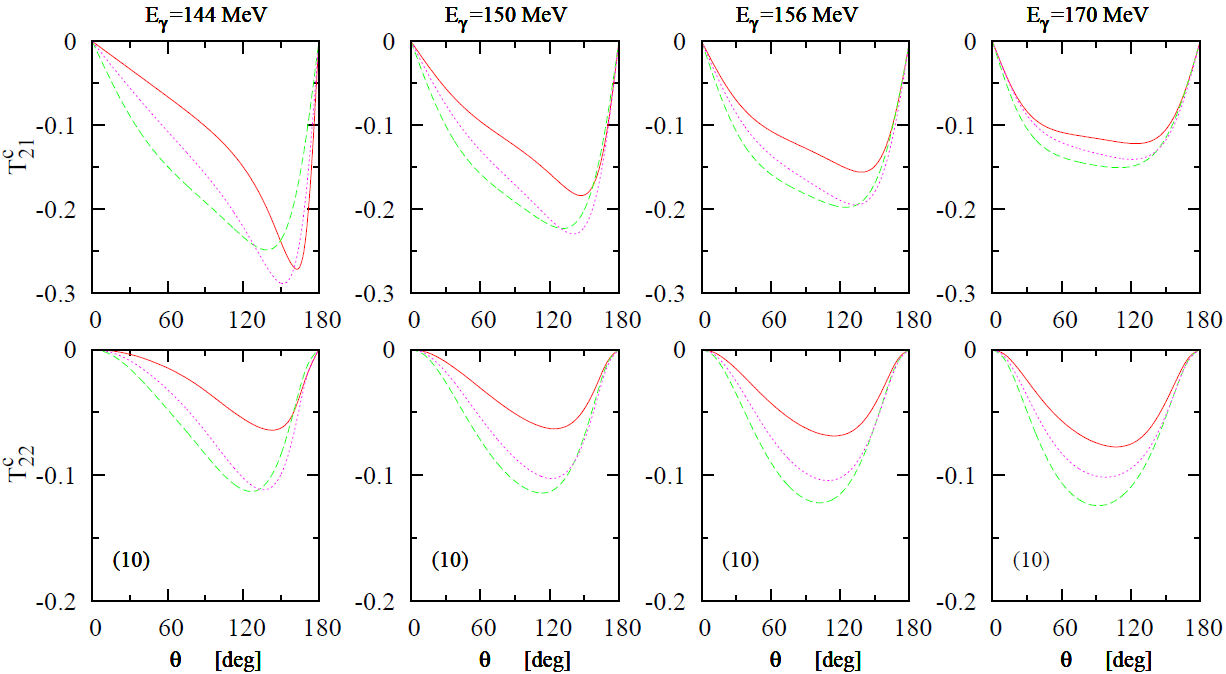
Figure 7 (Color online) Same as in Fig. 2 but for the beam-target double-spin asymmetries with circularly polarized photons and tensor polarized deuterons. Results for the
In Fig. 8 we present our results for
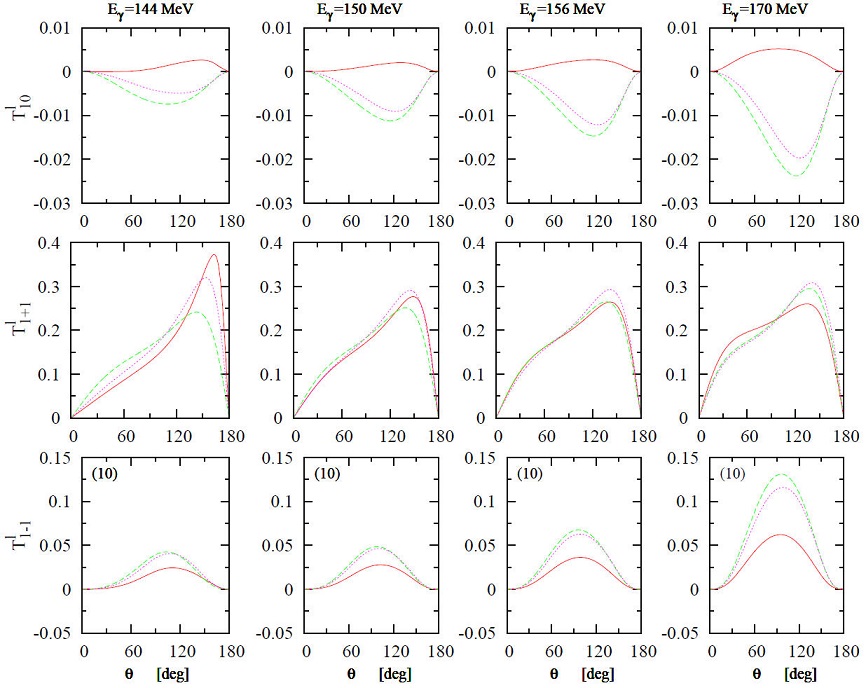
Figure 8 (Color online) Same as in Fig. 2 but for the beam-target double-spin asymmetries with linearly polarized photons and vector polarized deuterons. Results for the
The results for the beam-target double spin asymmetries
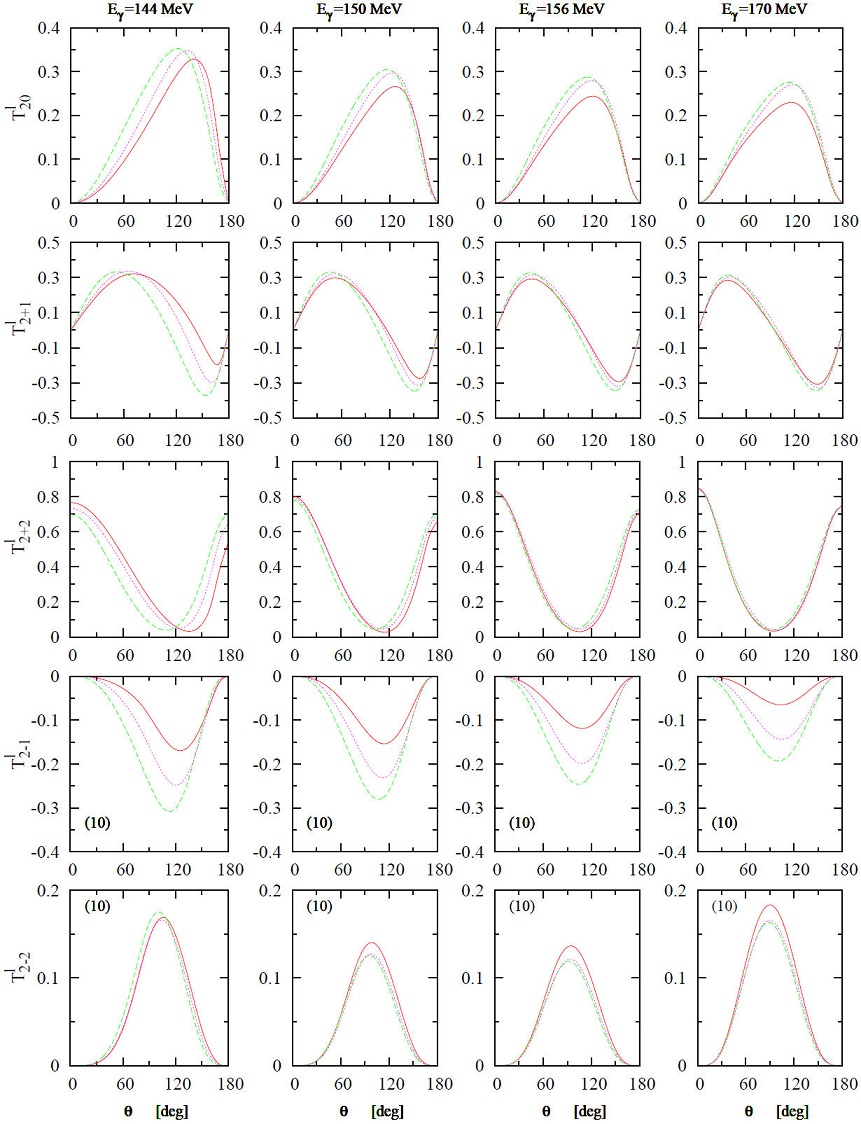
Figure 9 (Color online) Same as in Fig. 2 but for the beam-target double-spin asymmetries with linearly polarized photons and tensor polarized deuterons. Results for the
Figure 10 shows the results for the beam-target double-spin asymmetries

Figure 10 (Color online) Same as in Fig. 3 but for the beam-target double-spin asymmetries
3.4 Helicity-dependent cross sections and E-asymmetry
Next, we present in Fig. 11 the results for the doubly polarized differential cross sections for parallel (upper part) and antiparallel (middle part) spins of photon and deuteron as functions of pion angle in the c.m. frame at various photon lab-energies using different elementary amplitudes. These polarized differential cross sections are given by

Figure 11 (Color online) Same as in Fig. 2 but for the doubly polarized differential cross sections for parallel (upper part) and antiparallel (middle part) spins of photon and deuteron and their difference (lower part). Results at Eγ = 144 MeV are multiplied by the factor in the parentheses.
In Fig. 11 we also present an important physical observable which is the difference d(σP — σA)/dΩ (lower part) that has a direct relation with the GDH sum rule [49]. The results displayed show the contribution of each dσP/dΩ and dσA/dΩ on the difference. Since their values have opposite behavior with increasing θ, we can see that the difference has negative values at small pion angles 0o < θ < 30o because of dσA/dΩ has the larger contribution in this range of pion angles. Thereafter, for θ ranges between 30o and 90o, d(σP — σA)/dΩ starts to increase and behaves the same as dσP/dΩ. For θ = 90o, the values of d(σP — σA)/dΩ start to decrease due to dσA/dΩ which has larger contribution again.
We would like to mention that the threshold region is dominated by the pion production with spin 1/2, which makes the antiparallel term dσA/dΩ larger than the parallel one dσP/dΩ. The estimated values of d(σP — σA)/dΩ close to threshold at Eγ = 144MeV are rather small compared to their values at higher energies under consideration.
As for the dσP/dΩ results (upper part in Fig. 11), we observe that the results are sensitive to the choice of elementary amplitude at the peak region since we obtain smaller values using MAID-2007 than using DMT-2001 and χMAID-2013. At extreme forward and backward pion angles, similar results are obtained and the sensitivity of dσP/dΩ results to the elementary amplitude is negligible. In the case of dσA/dΩ results (middle part in Fig. 11), we see that the influence of dσA/dΩ on the elementary amplitude is similar to the case of unpolarized differential cross section. One can see that the differences among dσA/dΩ results using different elementary amplitudes are very obvious at forward pion angles and the calculation within the MAID-2007 is smaller than those within DMT-2001 and χMAID-2013 but provides similar results at extreme pion backward angles. This discrepancy shows up the differences among elementary amplitudes. The computations of the differential spin asymmetry with respect to circularly polarized photons and oriented deuterons, d(σP — σA)/dΩ, (lower part in Fig. 11) shows that the sensitivity to the choice of elementary amplitude is also important in the peak position. This means that the difference d(σP — σA)/dΩ is also sensitive to the choice of the elementary amplitude.
Figure 12 shows the results for the helicity-dependent total cross sections for the

Figure 12 (Color online) Same as in Fig. 3 but for the doubly polarized total cross sections for parallel (left panel) and antiparallel (middle panel) spins of photon and deuteron and their difference (right panel).
During the recent years, there is a considerable interest in experiments [1-7] to measure the double polarization observable E for the
In Fig. 13 we present the results for
E-asymmetry as a function of the emission pion angle
θ in the γd c.m.frame at four fixed values
of the incident photon lab-energy. We see that the E-asymmetry
has qualitatively a similar behavior for all incident photon lab-energies
considered. Its maximum equals unity at θ = 0o and
180o. The curves begin with unity and decrease as the pion angle
increases until a minimum value at θ ≃ 120o is
reached. Then, it increases again to unity. The minimum value is shifted towards
lower pion angles with increasing Eγ. The negative
values in the E-asymmetry come mainly from higher positive
contribution in dσP/dΩ. As
mentioned in Refs. [22,34], the beam-target double polarization
E-asymmetry is an excellent observable to test any weakness
in the underlying elementary pion photoproduction model. Figure 13 shows that the helicity
E-asymmetry is sensitive to the choice of the elementary
3.5 Comparison with experimental data
We close this section by comparing our results with the available experimental data. In fact, several experiments to measure both single and double polarization observables for coherent π0-photoproduction on the deuteron are presently underway. Unfortunately, no data are currently available for these polarization observables in the kinematic region under consideration in the present work and therefore we cannot make a comparison to experimental data for polarization observables. Thus, in the present work we compare the calculated results for the unpolarized differential cross section with the available data.
Figure 14 shows a comparison between our results for the unpolarized differential cross section dσ0/dΩ at two values of photon lab-energies (Eγ = 151.4 and 171.8 MeV) and the experimental data from TAPS [4]. One readily sees, that the estimated results for dσ0/dΩ using different elementary amplitudes underestimate the experimental data at backward pion angles. The results with the χMAID-2013 model (solid curve) are the nearest one to the experimental data in this case. At forward pion angles and Eγ = 151.4 MeV, an overestimation of the results using χMAID-2013 and DMT-2001 elementary amplitudes is found. But the results for dσ0/dΩ using MAID-2007 model underestimate the last three data points at high angles. At Eγ =171.8 MeV and for forward pion angles, a good agreement with the experimental data is also obtained. In this case, the shape given by MAID model is consistent with data lacking only in strength. We would like to mention that the elementary operators considered in the present work use some values of resonance photocouplings which especially in case of neutron are not yet well understood, and fitting these values to the data could improve the agreement between theory and experiment.

Figure 14 (Color online) The unpolarized differential cross section for
We would like to point out that even at the PWIA level studied in the present work, there are also uncertainties due to the deuteron wave function, since one is involving large momentum components of the deuteron. Therefore, in Fig. 15 we present results for dσ0/dΩ using different deuteron wave functions in comparison with the experimental data from TAPS [4]. For this purpose, we use the deuteron wave functions of the Bonn full [55] (solid curve), CD-Bonn [56] (dashed curve), and Paris [57] (dotted curve) NN potentials. These potentials are exceedingly employed for numerical estimations of electromagnetic reactions on the deuteron, give a precise characterization of the NN scattering data and phase shifts, and used to characterize the range of the NN interaction.

Figure 15 (Color online) The unpolarized differential cross section for
It is clear from Fig. 15 that the results using the
deuteron wave function of the Paris potential is smaller than those using
CD-Bonn and Bonn full potentials. At Eγ = 151.4 MeV,
we note an overestimation of the results using CD-Bonn and Bonn full potentials
at θ < 60o, whereas a good agreement with the
experimental data using the Paris potential is obtained in this case. On the
contrary, a good agreement between the results using CD-Bonn and Bonn full
potentials and the experimental data is obtained at
Eγ = 171.8 MeV and forward pion angles. The
results using the Paris potential underestimate the experimental data in this
case. At backward pion angles, we see that the results using various
NN potentials underestimate the experimental data. The
origin of the differences obtained using various realistic deuteron wave
functions maybe due to the tensor force between two nucleons. It is well-known
that a practical measure for the strength of the tensor-force component
contained in a nuclear potential is the predicted D-state
probability of the deuteron, PD (see, for example,
Refs. [58,59]). The PD
values for the NN potentials used in this work are 4.85% for
CD-Bonn, 4.25% for Bonn full, and 5.77% for Paris. The difference in these
values of PD is related to the different tensor part
of the NN potentials. The dependence of the
The obtained discrepancies between our estimations for
dσ0/dΩ and the experimental
data can be attributed to the neglected contributions from two-body effects in
the transition
From the preceding discussions it is apparent that the choice of the elementary amplitude has a visible effect on unpolarized cross sections as well as on various beam, target, and beam-target spin asymmetries in the
4 Summary and outlook
The main topic of this article was to discuss theoretical uncertainties in the analyses of
We have found that the estimations of the uncertainty on the
The results presented above highlight the sensitivity of
In summary, we conclude that the calculated results are of particular interest for the evaluation of the systematic uncertainties caused by the use of different elementary operators in the analyses of