1 Introduction
Proton-projectile is one of the well-known nuclear probes. Using the proton as a projectile, we could obtain useful information about the nuclear structure and nuclear interaction. As an example, we could obtain information about the radial distribution of proton, neutron, and nuclear matter inside the nucleus. Moreover, from the proton-nucleus scattering, we could test the reliability of any theoretical model for nuclear structure or interaction.
Systematic studies on proton scattering from light weakly bound He, Li, and Be isotopes were carried out at wide energy ranges [1,2,3,4,5,6,7,8,9]. Polarized proton scattering from nuclei adds another constraint to the scattering problem and reflects various aspects of nuclear structures and reaction mechanisms. For example, Sakaguchi et al. [10] used the polarized proton for scattering from 6He to find the appropriate structure of this exotic nucleus. Uesaka et al. 11], found through analyzing Sakaguchi’s et al. data that dσ/dΩ favor the existence of 6He two neutrons halo at backward angles and that the cluster structure reproduced reasonably well the experimental data. In addition, they refered to the indirect effect of neutrons halo on Ay calculation. Mahmoud et al. [12] reanalyzed the dσ/dΩ and A y for the p+6He system in the framework of the optical model potential using microscopically real central and spin orbit (SO) potentials. They used CDM3Y6 energy and density-dependent version of the effective M3Y nucleon-nucleon (NN) interaction [13]. Their SO potential was calculated using the formalism reported in [13]. They aimed to study the validity of the microscopic SO potential based on CDM3Y6 effective NN interaction. In addition, they aimed to study the structure effect on the scattering observable for p + halo-nuclei system. As expected from these studies, the scattering of polarized protons shows different behavior in exotic nuclei compared to stable ones.
Many theoretical models were conducted to describe nuclear clustering in the 9Be [14,15]. The exotic 9Be nucleus attracted attention because of its Borromean structure and its cluster breakup. For four decades, the elastic scattering of protons from 9Be at low energies was extensively studied both experimentally and theoretically. Farag et al. [16] analyzed proton elastic scattering observables of 9,10,11,12Be at a wide range of energy between 3 and 200 MeV using the optical model. They calculated dσ/dΩ and A y and reaction cross sections σR using single folding (SF) real potential based on the density and iso-spin dependent M3Y effective NN interaction and imaginary part based on the high energy approximation. They used the Thomas form with a radial form for the SO potential based on the real folded potential. They claimed that the SF potential reproduced the scattering observables for energies up to 100 MeV using the non-relativistic Schrodinger equation. For higher energies, they found that the high energy approximation or the eikonal approximation could reproduce the scattering observables better than the optical model of the non-relativistic Schrödinger equation. Meridi [17] analyzed the elastic scattering of protons from 9Be nucleus at incident energies up to 1000 MeV/nucleon. He used energy-dependent microscopic optical model potential based on the density- and isospin-dependent M3Y-Paris nucleon-nucleon (NN) interaction for the real and spin-orbit parts. For the imaginary part, he used the NN-scattering amplitude of the high-energy approximation. His microscopic complex spin-orbit was taken within Breiva-Rook approximation [18]. He found that the optical model potential fails to reproduce the differential cross-section data at energies larger than 100 MeV/nucleon. In addition, he found that a good improvement is obtained by including the surface contribution to the imaginary part. Recently, Maridi et al. [19] analyzed elastic scattering data for p+9Be at proton incident energy below 30 MeV by using two techniques. Their two techniques lead to similar normalized values for the existing data and consistently validate that low-energy data to be safely used for further theoretical studies.
Bingham et al. [20] measured the p+9Be scattering differential cross sections at eleven bombarding energies between 5 and 15.1 MeV. Their data cover a wide angular range from 15° to 170° in the center of mass (c.m.) system. These low energy data were found to differ by 15-20% between different measurements [21]. These data [20] were investigated by Keely et al. [21] to trace and remove any normalization inconsistencies using a coherent coupled reaction channels (CRC) method. The results of Keely et al. [21] motivated Pakou et al. 9] to reanalyze the data for this system using the microscopic JLM complex potential. The results of Keeley [21] and Pakou[9] support the conclusion of negligible or no compound elastic contribution to the elastic scattering dσ/dΩ at low energies.
The measured data of the elastic scattering Ay in conjunction with available dσ/dΩ of protons by complex nuclei extended the scope of the optical model. The analysis of A y data is essential and unique in obtaining information about the nuclear SO interaction. The availability of dσ/dΩ data makes the central parts of the optical model well defined where the SO potential has only a small effect upon the calculated dσ/dΩ. So, A y measurements and analysis are required to determine the SO interaction in a systematic way [22].
The results of Refs. [12,21,9] motivated us to reanalyze the elastic scattering of the proton from the exotic 9Be. The aim is to study the applicability of the microscopic SO potential based on the CDM3Y6 effective NN interaction for this system. In addition, we aimed to examine the success of the central SF real potential for analyzing scattering data at the considered low energy.
2 Theoretical formalism
2.1 Central real potential
In the present work, we used the SF model to calculate the p+9Be central real potential. This real central potential is calculated through the folding procedure from the following relation,
As shown from Eq. (1), the SF model has two essential ingredients: 1) a realistic NN effective interaction and, 2) target point nucleon density distribution. The energy- and density-dependent CDM3Y6 effective NN interaction [23] is used as an effective NN interaction ,
where the intrinsic energy g(E) and density F(ρ) dependent factors [13] have the following forms,
The radial forms of the iso-scalar (isospin T = 0) and iso-vector (isospin T = 1) components of the central M3Y-Paris interaction [13] have the following Yukawa forms,
Similarly, the SO components are represented in the Yukawa forms as,
The explicit ranges and strengths parameters of these Yukawa forms as given by Khoa et al. [13] are presented in Table I. The direct part of the real central folded potential is computed from,
Table I Yukawa ranges and strengths of the central and SO components of the M3Y-Paris effective NN interaction.
i | Ri fm-1 |
|
|
|
|
|
|
---|---|---|---|---|---|---|---|
1 | 4.0 | 11061.625 | 313.625 | -1524.25 | -4118.0 | -5101.0 | -1897.0 |
2 | 2.5 | -2537.5 | 223.5 | -518.75 | 1054.75 | -337.0 | -632.0 |
3 | 0.7072 | 0.0 | 0.0 | -7.8474 | 2.6157 | 0.0 | 0.0 |
The exchange part of the real central folded potential is computed from,
j0(x) is the zero order spherical Bessel function. ρ0,1(r) are the iso-scaler and iso-vector densities, respectively, which are defined as,
and k(E,R) is the relative momentum, which has the form,
Here M stands for the reduced nucleon mass, VC (R) is the Coulomb potential and V(E, R) is the total real folded nuclear potential. The density matrix ρk (R, r), (k = p, n), is considered using the following approximation,
Where p, n stand for proton and neutron, respectively.
2.2 Central SO potential
The formalism described in an earlier report[13] is used to calculate the SO-potential in the present work. According to this formalism and using the SO component of CDM3Y6 effective NN interaction and the target nuclear matter density the SO potential is computed microscopically as,
For comparison, we used a Thomas form SO potential with radial form factor based on WS or the SF real central potentials, respectively. Thus the SO potentials used in this work are written formally as,
This density form is known as a modified Gaussian and has charge root-mean-square radius
3 Results and discussion
We used the auto-search optical model computer code HERMES [26] to optimize our calculated elastic scattering dσ/dΩ and A
y to the experimental data. The optimization are carried out by minimizing the
where,
W0, Ri and ai are the depth and shape parameters of the imaginary potential, respectively. In the optimization procedure, we searched for optimizing Nr, Nso and the WS shape parameters for surface imaginary and Thomas form SO potentials, respectively. Our calculated elastic scattering dσ/dΩ and Ay are shown in Figs. 1, 2. The optical model best fit parameters and the corresponding calculated quantities are listed in Tables II-IV.

Figure 1 Calculated dσ/dΩ (left panels) and Ay (right panels) for p+9Be system at energies between 3 and 7 MeV.

Figure 2 Calculated dσ/dΩ (left panels) and Ay (right panels) for p+9Be system at energies between 8 and 15 MeV.
Table II Phenomenological optical model fitting parameters for p+9Be system at energies between 3 and 15 MeV.
Ep MeV | Nr | W0 MeV | ri fm | ai fm | V0oso MeV | rso fm | aso fm |
---|---|---|---|---|---|---|---|
3 | 1.362 | 4.365 | 2.512 | 0.497 | 5.719 | 1.736 | 0.114 |
5 | 1.163 | 7.218 | 1.674 | 0.511 | 10.403 | 1.362 | 0.104 |
6 | 1.198 | 10.732 | 1.399 | 0.511 | 9.635 | 1.282 | 0.106 |
7 | 1.157 | 11.484 | 1.509 | 0.513 | 8.471 | 1.302 | 0.104 |
8 | 1.116 | 9.926 | 1.596 | 0.509 | 7.252 | 1.372 | 0.200 |
9 | 1.081 | 8.788 | 1.423 | 0.550 | 7.225 | 1.378 | 0.190 |
10 | 1.048 | 10.080 | 1.368 | 0.553 | 4.564 | 1.211 | 0.158 |
15 | 1.027 | 7.496 | 1.273 | 0.552 | 3.833 | 1.164 | 0.152 |
Table III Optical model fitting parameters for p+9Be system at energies between 3 and 15 MeV.
EpMeV | Potential | Nr | Nso a | W0 MeV | ri fm | ai fm |
---|---|---|---|---|---|---|
3 | MI-SO | 1.342 | 1.143 | 4.220 | 2.600 | 0.526 |
CE-SO | 1.336 | 0.277 | 4.035 | 2.600 | 0.540 | |
5 | MI-SO | 1.224 | 1.555 | 7.330 | 1.725 | 0.511 |
CE-SO | 1.211 | 0.328 | 8.095 | 1.741 | 0.511 | |
6 | MI-SO | 1.135 | 1.345 | 11.034 | 1.607 | 0.533 |
CE-SO | 1.112 | 0.245 | 12.631 | 1.589 | 0.533 | |
7 | MI-SO | 1.067 | 1.550 | 13.140 | 1.567 | 0.503 |
CE-SO | 1.097 | 0.301 | 14.392 | 1.539 | 0.503 | |
8 | MI-SO | 1.065 | 1.242 | 8.530 | 1.210 | 0.545 |
CE-SO | 1.085 | 0.255 | 10.746 | 1.572 | 0.505 | |
9 | MI-SO | 1.070 | 1.409 | 7.731 | 1.164 | 0.560 |
CE-SO | 1.169 | 0.247 | 14.112 | 1.117 | 0.508 | |
10 | MI-SO | 1.134 | 1.405 | 12.946 | 1.126 | 0.550 |
CE-SO | 1.115 | 0.260 | 13.565 | 1.078 | 0.550 | |
15 | MI-SO | 1.056 | 1.200 | 10.764 | 1.141 | 0.540 |
CE-SO | 1.078 | 0.275 | 10.630 | 1.128 | 0.556 |
Table IV Volume integrals for central real, central imaginary and SO potentials.
Ep MeV | Potential | Jr MeV·fm3 | Ji MeV·fm3 | Jso MeV·fm3 |
|
---|---|---|---|---|---|
3 | PH-SO | 855.4 | 340.5 | 57.67 | 992.3 |
MI-SO | 843.1 | 373.7 | 40.26 | 1027.0 | |
CE-SO | 839.6 | 367.7 | 50.90 | 1033.0 | |
5 | PH-SO | 719.1 | 267.4 | 82.30 | 659.0 |
MI-SO | 756.7 | 287.3 | 54.24 | 791.9 | |
CE-SO | 748.5 | 322.8 | 58.87 | 820.2 | |
6 | PH-SO | 734.5 | 285.6 | 71.75 | 676.9 |
MI-SO | 696.0 | 397.8 | 46.68 | 818.9 | |
CE-SO | 681.9 | 446.0 | 44.02 | 817.4 | |
7 | PH-SO | 703.8 | 352.8 | 64.07 | 730.6 |
MI-SO | 649.0 | 422.9 | 53.53 | 784.6 | |
CE-SO | 667.3 | 447.7 | 53.68 | 779.5 | |
8 | PH-SO | 673.7 | 335.2 | 57.79 | 749.6 |
MI-SO | 642.6 | 189.6 | 42.68 | 717.0 | |
CE-SO | 654.7 | 349.7 | 45.14 | 774.4 | |
9 | PH-SO | 647.2 | 263.5 | 57.85 | 707.0 |
MI-SO | 640.4 | 166.5 | 48.18 | 671.6 | |
CE-SO | 699.6 | 250.0 | 43.39 | 718.8 | |
10 | PH-SO | 622.3 | 283.4 | 32.11 | 724.8 |
MI-SO | 673.2 | 257.6 | 47.8 | 705.4 | |
CE-SO | 662.0 | 250.5 | 45.34 | 709.1 | |
15 | PH-SO | 586.1 | 185.0 | 25.92 | 572.3 |
MI-SO | 602.4 | 213.8 | 39.85 | 599.3 | |
CE-SO | 615.0 | 215.0 | 46.19 | 611.0 |
In Fig. 1 we present the calculated dσ/dΩ (left panels) and A y (right panels) for p+9Be system at energies between 3 and 7 MeV. As shown from this figure, the calculated Ph-, MI- and CE-SO potentials are able to reproduce the experimental dσ/dΩ with equal success. For A y the MI- and CE-SO potentials failed to reproduce the experimental data successfully but have the same angular distribution pattern. The PH-SO potential is successfully reproduced both the dσ/dΩ and A y angular distributions for all the considered energies.
Moreover, we found that the success of MI-SO and CE-SO potentials are improved in reproducing A y angular distributions as energy increases.
Our calculated dσ/dΩ (left panels) and A
y
(right panels) at energies between 8 and 15 MeV are shown in Fig. 2. As shown in this figure, the MI- and CE-SO potentials reproduced both the dσ/dΩ and A
y
reasonably well. The improvement of the calculated A
y
started at 8 MeV, where both MI- and CE-SO potentials reproduced the angular distribution successfully at the foreword angles up to
The energy dependence of optical model searched parameters, and calculated quantities are shown in Figs. 3, 4. From Fig. 3, we see that the real J r for the three potentials globally decrease exponential-like with increasing energy. A fine look at this figure shows that the N r has a small hill at 5, 10, and 9 MeV for Ph-, MI- and CE-SO potentials, respectively. The energy dependence of J i has a peak at around 6.5 MeV for MI- and CE-SO and at 7 MeV for PH-SO potentials. Moreover, MI-SO potential has a minimum of around 8.5 MeV.
The corresponding
In Fig. 5, we present the energy dependence of the total reaction cross sections
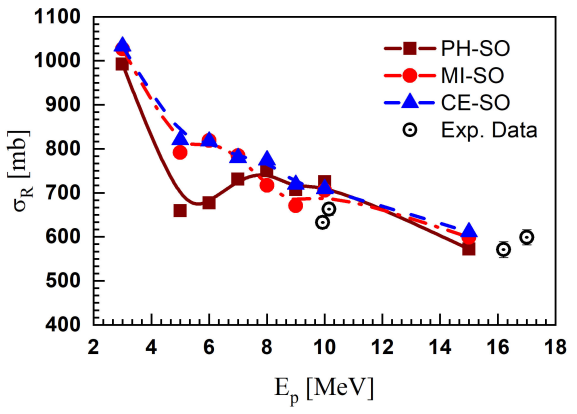
Figure 5 Calculated total reaction cross sections
From these energy dependences, we could expect the influence of 9Be breakup channel at the energy interval between 5-8 MeV. In addition, we could conclude that the present data are not entirely free from the normalization problem. This conclusion comes from the data analysis at 5 and 6 MeV, where a theory normalization for PH-SO calculation 1.276, 1.123 has to be introduced to reproduce the data, respectively.
4 Conclusion
In the present work, we analyzed the p+9Be elastic scattering at 3-15 MeV. Both the differential dσ/dΩ and A y are analyzed simultaneously in the framework of the optical model. The real part of the optical model potential is computed using the SF procedure. For the SO potential, we adopted microscopic and phenomenological Thomas form methods. For microscopic SF and SO potentials, the CDM3Y6 effective NN interaction is used. In the phenomenological Thomas form method for the SO potential, the radial form factor is chosen in the WS-form (phenomenological form) or in the form of the calculated SF real potential (semi-microscopic form). The optical potential imaginary part is adopted in the conventional surface WS form throughout this analysis.
The optimization of the calculated dσ/dΩ and A y is done using the spherical optical model code HERMES [26]. We found that the SF real potential with the different versions of SO potentials can reproduce the dσ/dΩ and the A y with the phenomenological SO potential for all energies. Also, we found that the microscopic SO potential and the semi-microscopic SO potentials cannot reproduce the A y at energies below 8 MeV, while they are reasonably and successfully reproduced A y at energies ≥ 8 MeV. The success of this microscopic SO potential is increased while increasing energy.
In conclusion, we found that the microscopic SO potential is successful in reproducing the analyzing power A y for the p+9Be system contrary to the founding of p+6He [12]. The present analysis provides a good application for using the microscopic spin-orbit potential. The success of the microscopic SO potential motivates us to study the structural effects of 9Be on the scattering observable and other calculated quantities. Also, it encourages us to extend it to other nuclei where experimental A y data exist over a wide energy range.