INTRODUCTION
The concept that certain disease states can damage homeostatic organs, such as the kidneys, resulting in the accumulation of harmful molecules in the blood (toxins solutes [TS]) and excess fluids is well-established. This understanding has led to the development of blood purification therapies, including hemodialysis (HD) and hemoperfusion (HP)1,2.
As an example, reduced kidney function in uremic patients can result in the accumulation of various TS, including peptides, proteins, and protein-bound compounds, in the blood3. The increased levels of these TS have been linked to specific clinical outcomes4,5. It also has been well established that the accumulation of such TS in the plasma is associated with cardiovascular risk, chronic kidney disease progression, bone mineral disorders, neurologic manifestations, and inflammation, significantly contributing to poor quality of life and high mortality in maintenance HD patients (Fig. 1). According to the molecular weight (MW), TS are classified into six classes, including small water-soluble molecules (< 500 Da), protein-bound uremic toxins (PBUTs; mostly < 500 Da), small-middle molecules (0.5–15 kDa), medium-middle molecules (15–25 kDa), large-middle molecules (25–58 kDa), and large molecules (> 58 kDa)3. While some TS are very large (51 kDa) and require plasma exchange for removal, others are small enough to be eliminated through dialysis, filtration, or adsorption through sorbent beads6. Table 1 summarizes the main TS, their classification, representative biomarkers, relevant clinical effects, and the expected extracorporeal modalities for removal.

Figure 1. Toxin Solutes and Relevant Clinical Effects. Toxin solutes commonly encountered in clinical settings can encompass a wide range of substances classified according to their molecular weight and protein binding. The clinical effects depicted highlight the diverse manifestations such as renal dysfunction, cardiovascular complications, neurological impairments, and other systemic effects. Created with BioRender.com.
Table 1. Toxin solute classification, representative biomarkers, relevant clinical effects, and expected extracorporeal modalities for removal
Toxin solutes class | Molecular weight (Da) |
Representative biomarkers | Relevant clinical effects | Extracorporeal modality expected to remove |
---|---|---|---|---|
Small water-soluble mulecules | < 500 Da | Urea (60 Da), creatinine (113 Da), phospate (94), uric acid (168 Da) | General uremic toxicity, Uremic symptoms, Endothelial dysfunction, Vascular calcification | All dialysis modalities |
Small protein-bound uremic toxins | Mostly < 500 Da | Indoxyl sulfate (213 Da), p-cresyl sulfate (210 Da) | General uremic toxicity, Inflammation, Oxidative Stress | All dialysis modalities |
Small-middle molecules | 0.5-15 kDa | PTH (9.5KDa), β2 Microglobulin (11.8 KDa) | Bone mineral disease, Vascular calcification, Dialysis-related amyloidosis | High Flux
HD HDF HDx HCO |
Medium-middle molecules | > 15-25 KDa | Myoglobin (17 kDa),TNF-α (17 kDa), IL-10 (18 kDa), κ-FLC (22.5 kDa), IL-18 (24 kDa), IL-6 (24.5 kDa) | Oxidative Stress, Mitochondrial, Dysfunction, Sepsis, Inflammation, Multiple toxicity | HDF HDx HCO |
Large-middle molecules | > 25-58 kDa | FGF-23 (32 kDa), VEGF (34.2 kDa), λ-FLC (45 kDa) | Cardiovascular complications, Inflammation | HDx HCO |
Large molecules | > 58-170 kDa | Modified albumin, Albumin (65 kDa) | Toxin Binding | HCO Plasmapheresis |
Da: Dalton; PTH: parathyroid hormone; TNF-α: tumor necrosis factor-alpha; IL: interleukin; FGF: fibroblast growth factor; κ-FLC: κ free light chains; VEGF: vascular endothelial growth factor; λ-FLC: λ free light chains; HD: hemodialysis; HDF: hemodiafiltration; HCO: high cut-off; HDx: expanded hemodialysis.
In clinical settings, blood purification techniques achieve molecular solute separation primarily by membrane-based (diffusion, convection, or both) and adsorptive processes (adsorption) (Fig. 2)7-9. The term dialysis refers to the exchange process that takes place between two compartments: the blood and dialysis fluid through a semipermeable membrane. This exchange can occur through a concentration gradient (diffusion) as in standard HD, hydrostatic pressure (convection) as in HF, or a combination of both as in hemodiafiltration (HDF). The membrane plays a crucial role in removing TS and excess water during this process. The properties of the membrane, including the nature of the material, structure, biophysical characteristics, pore size, permeability, and biocompatibility, are critical factors in determining the effectiveness and safety of the dialysis process. Therefore, membrane geometry is essential for optimizing solute mass exchange7.
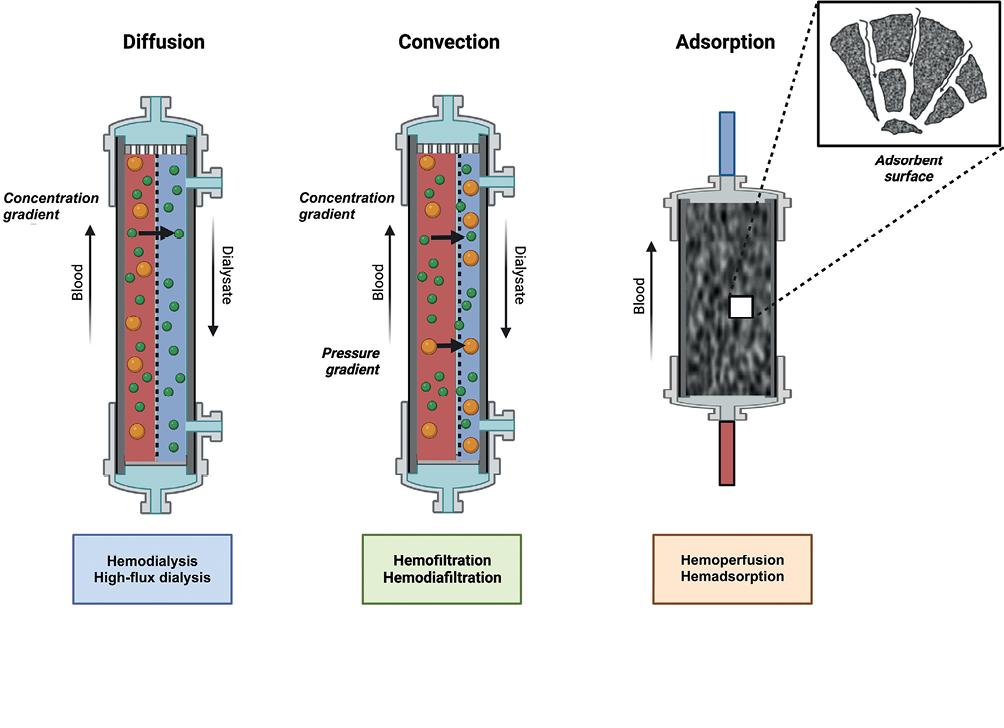
Figure 2. Mechanism of solute transport and blood purification modalities. Three mechanisms are currently used for blood purification in extracorporeal therapies: diffusion, convection, and adsorption. Diffusion and convection are membrane-based. Adsorption, instead, is a mass separation process by a solid agent (sorbent). These mechanisms of solute transport, both individually and in combination, form the basis for various modalities of blood purification. Created with BioRender.com.
Adsorption, instead, is a mass separation process by a solid agent (sorbent)9. Adsorption involves the interaction between adsorbent (material where adsorption takes place) and adsorbate (molecule adsorbed onto the surface of the adsorbent). It differs significantly from the classic mechanisms of transport based on separation by a barrier (dialysis membrane). Therefore, it can overcome the limitations imposed by dialysis membranes and effectively remove a broader spectrum of solutes1,9,10.
The development of newer membrane biomaterials and manufacturing has improved their characteristics and properties11 and led to reconsidering the classical membrane classification12. Advancements in HD membranes and sorbents have improved the efficacy and safety of these blood purification therapies. These innovations have enabled removing a wider range of toxic solutes, improving patient clinical outcomes.
FROM CELLOPHANE TO HIGH-FLUX: THE EVOLUTION OF HEMODIALYSIS MEMBRANES
The evolution of HD membranes, from the first cellophane membrane used to treat patients in the rotating drum kidney to the widespread use of hollow-fiber-high-flux membranes in the modern era, has been remarkable13. Figure 3 shows the development of the different HD membranes and sorbents in a timeline.
Earlier discoveries by Thomas Graham (1861) on the properties of semi-permeable vegetable membranes presented the principles of solute transport coining the term "dialysis" for the first time. At the beginning of the 20th century, semi-permeable membranes were produced using natural materials, mainly cellulose. The first proper artificial kidney was presented by Abel et al., (1913)14 followed of the first HD in a human being by George Hass. Together allowed the evolution to development and implementation of the rotating drum kidney by Kolff et al. in the 1940's15. The rotating drum kidney was the first HD clinical apparatus and was consisted of a 30 m cellophane tube (inner diameter = 35 mm) spirally wrapped around a cylinder. This cylinder rotated within a stationary dialysate bath. While a blood pump was not required due to the minimal resistance in the blood compartment, the system's low transmembrane pressures (TMPs) significantly restricted its ultrafiltration (UF) capabilities. Rotating drum kidney was latter perfectionated by Alwall.
Following the rotating drum kidney, the coil dialyzer16 and a commercial variant of this device, known as the "twin coil"17 (two coils with a combined surface area of 1.8 m2), gained widespread usage. In this dialyzer, cellophane tubing was encased by a fiberglass screen and arranged in a single coil within a large cylindrical drum of dialysate. This design increased blood compartment resistance, leading to elevated TMPs and significant obligatory UF volumes. Unfortunately, UF volumes could not be predicted or reliably controlled; therefore, convective therapies were only made possible until the development of UF control systems. Later, the Kiil dialyzer and its subsequent adaptations utilized a parallel blood-dialysate flow setup for the first time18.
All these membranes and devices were composed of natural cellulose, and were universally used until the early 1970s. The classical cellulose membrane family comprises two main categories: unsubstituted cellulose (cuprophane) and substituted cellulose (di-or tri cellulose acetate). These cellulosic membranes had small pore sizes and wall thickness ≤15 µm, which facilitated diffusion transport and provided efficient clearance of small water-soluble molecules, such as creatinine (MW: 113 Da) and urea (MW: 60 Da). However, due to their low flux and inherently low water permeability, convective transport and removal of larger molecules were limited. Over the years, the use of cellulosic membranes has been decreasing, which has practically been replaced by synthetic membranes13.
Hollow-fiber membranes
In the late 1960s, introducing the hollow-fiber artificial kidney19 brought significant advancements in blood rheology and solute mass transfer. Today, dialyzer membranes are almost exclusively manufactured as hollow fibers (e.g., capillary fiber). This configuration offers specific advantages, such as an enhanced surface-area-to-volume ratio in the blood compartment, resulting in shorter diffusion paths. In addition, it reduces boundary layer effects while maintaining acceptable pressure drops. Consequently, the hollow-fiber design optimizes solute exchange between blood and dialysate fluid20. Hollow-fiber hemodialyzers are assembled in a cylindrical bundle (≈ 10-20,000 fibers); the assembly is then enclosed in a polymeric cylindric case housing potted at both ends and open onto the two heads (artery and venous ports) of the dialyzer in a distribution chamber21. At present, hollow-fiber hemodialyzers are the main dialyzers utilized in HD worldwide.
By the end of the 20th century, synthetic membranes made from polymers were developed. The initial synthetic membranes had very thick walls, ≈ 100 µm, which made them unsuitable for diffusion-based treatments. As a result, they were initially limited to HF, which relies solely on convection. Modern hollow-fiber synthetic membranes are made from polymers such as polysulfone (PSU), polyethersulfone, polyacrylonitrile including sulfonated polyacrylonitrile (AN69), and poly (methyl methacrylate) (PMMA). Over time, advances were made by significantly reducing the wall thickness, and nowadays, they typically have a wall thickness ranging from 20 to 50 µm and high permeability, which facilitates diffusion and convective transport, enabling synthetic membranes to be used for high-flux HD and HDF. AN69 and PMMA membranes have a symmetric structure, where the composition remains consistent throughout the entire wall thickness, where the diffusive process takes place all over the length of the wall thickness (40–50 µm). However, most synthetic membranes possess an asymmetric structure that consists of a thin inner layer, so-called thin skin (with a width of approximately ≤1 µm) that plays the primary role in selectively removing solutes (sieving layer). The remaining membrane thickness (40–50 µm), called the "stroma," serves as a support structure and provides a large surface area for molecules to be removed through adsorption. The stroma has a relatively open structure, often described as "macroporous," and typically exhibits a sponge-like or finger-like arrangement. Figure 4 shows the three-layer structure of hollow fiber membranes,

Figure 4. Hollow fiber membranes structure. The three-layer structure of hollow fiber membranes used in blood purification consists of a thin inner layer (skin), a middle layer (stroma), and an outer layer (coating layer). The intricate design allows for effective separation of solutes during membrane-based extracorporeal therapies.
Synthetic polymer membranes often need a copolymer blend (e.g., polyvinylpyrrolidone [PVP]) to enhance hydrophilicity, alter surface properties, improve blood compatibility, prevent surface fouling, and adjust solute permeability. Developing newer membrane biomaterials and manufacturing processes, such as polymer blending and surface functionalization, has led to considering several other parameters for membrane categorization, including new permeability indices, hydrophilic versus hydrophobic balance, adsorption capacity, and electrical potential11.
Dialysis membranes have been broadly classified on their composition (cellulosic or non-cellulosic), small-solute clearance capabilities (low or high-efficiency), permeability (low flux and high flux), or very high permeability (super flux, so-called medium, high cutoff or protein-leaking membranes)7. Assessing a dialysis membrane's performance involves its permeability profile; membrane permeability should be characterized by hydraulic (UF coefficient) and solute permeability (solute mass transfer coefficient for low MW or sieving coefficient for middle or large MW). Compared with low-flux HD membranes, high-flux membranes have a higher UF coefficient, resulting from increased hydraulic permeability. They also have larger pores that maximize the clearance of middle MW solutes, including β2- microglobulin (β2M). However, convection (flux) is the most critical determinant of β2M removal. Comparisons of physical characteristics of the membrane structure are shown in table 2.
Table 2. Comparison of physical characteristics of hemodialysis membrane structures
Hemodialysis Membrane | Molecular weight cut-off (kDa) | Mean pore radios (μm) | Fiber inner diameter (μm) | Wall thickness (μm) | Pore size distribution | Ultrafiltration coefficient (ml/h/mmHg) | Sieving coefficient (β2-microglobulin) |
---|---|---|---|---|---|---|---|
Low Flux | < 15 | 2-4 | 200 | 5-15 | Narrow | < 20 | < 0.01 |
High Flux | Hemodialysis:
25 Hemodiafiltration:30 |
4 | 200 | 20-50 | Wide | > 50 | > 0.9 |
Medium cut-off | 55 | 5 | 180 | 35 | Wide/Tight | > 50 | > 0.9 |
High cut-off | > 55 | > 8 | 215 | 50 | Wide | > 50 | > 0.9 |
Please note that the values provided are approximate ranges and can vary depending on the specific manufacturer and type of hemodialysis membrane.
The application of convective therapies such as high-flux HD, HF, and HDF was not possible until the advent of machines with UF control systems 25 years ago. Gradually high-flux HD became the most common mode of HD treatment worldwide. High-flux membranes have made the application of convective therapies, such as HF and HDF, possible. Convective and diffusive solute transportation is achieved in HDF by applying a high-flux membrane, countercurrent dialysis fluid, and ultrapure (sterile non-pyrogenic) replacement fluid into the venous flow; so-called online HDF (ol-HDF). In HDF modality, molecules up to 30 kDa are efficiently removed. The superiority of ol-HDF compared with high-flux HD on solute removal of middle and large MW has been proven. A prospective, cross-sectional study comparing high-flux HDF versus super-flux HD was performed in 21 patients. Percentage reduction of urea, creatinine, β2-microglobulin, myoglobin, prolactin, a1-microglobulin, and a-1 AGP and albumin loss were evaluated. The B2-microglobulin, myoglobin and prolactin reduction were significantly higher with HDF. There were no significant differences in albumin loss between treatments . The study confirms the superiority of post-dilution HDF with less albumin loss22.
However, there has been a debate about the superiority of ol-HDF compared with high-flux HD on survival benefits, and previous studies were inconclusive23-25. Until very recently, only one study showed a survival benefit for HDF26. At least >23 L/session (High dose HDF) of convective clearance is required in ol-HDF to achieve the survival benefits over standard HD27. Recently, a multicenter study of prevalent high-flux HD patients that were randomized to high-dose HDF (>23 L/session) versus continuing on high-flux HD showed a reduced risk of death with HDF compared to conventional high-flux HD (17.3% vs. 21.9 %, HR, 0.77, 95% CI, 0.65-0.93)28. There is some practice preference for HD overall; nonetheless, HDF is now applied across continents, especially in Europe and Asia, but also in Latin American countries such as Mexico with online production of replacement fluid (ol-HDF), allowing achievement of convective volumes up to 25 L/session.
ADVANCES IN IMPROVING THE BIOCOMPATIBILITY OF MEMBRANES
Progress in dialysis membranes can be divided into two categories: enhancements in membrane performance (e.g., solute permeability) and advancements in membrane biocompatibility. Membrane characteristics are crucial for controlling solute mass transfer and modulating blood membrane interaction bioreactivity. Biocompatibility advancements aim to minimize biological and inflammatory reactions when blood components interact with the dialysis membrane and the blood circuit29. A biocompatible or "inert" membrane minimizes biological and inflammatory responses on exposure30. Biological reactions can occur due to various factors, including membrane nature, composition (cellulosic or polymer), surface characteristics, and unique features of the membrane or extracorporeal circuit. The dialysate fluid's composition and purity also influence mediators' release. Consequently, determining the specific role of the membrane in biocompatibility is challenging due to the simultaneous occurrence and interaction of multiple factors.
Relative biocompatibility was associated with the unmodified cellulose membranes used in early dialysis applications31-33. However, this is no longer a concern as synthetic membranes have universally replaced them.
There have been reports of hypersensitivity-like reactions, specifically Type A reactions, associated with membranes based on PVP. The exact mechanism is still unknown but complement activation has been suggested as a potential cause in patients sensitized to specific components of the dialysis materials, particularly PVP. PVP is commonly used as a hydrophilic additive in blending with PSU to modify its hydrophobic properties. The PSU/PVP blend exhibits interesting characteristics, including increased hydraulic and solute permeability and improved hemocompatibility by creating a domain-like surface structure with hydrophilic and lipophilic regions on the blood-membrane interface34. PVP is leached from synthetic membranes, especially under specific sterilization conditions like gamma-ray radiation33,35. Furthermore, PVP leaching might be a potential cause of an anaphylactic reaction in a HD patient; reports suggest that increased membrane permeability during bleach-based reprocessing of high-flux PSU dialyzers is due to PVP leaching36. However, conclusive results have not been obtained so far. Nonetheless, the reported incidence of dialysis-related adverse events remains rare (0.17-4/1000 sessions per year)37,38.
In the past, dialyzers were typically made from polycarbonate. Recently, new polymers like polypropylene and polystyrene were introduced to prevent bisphenol A (BPA) release35,39. BPA is a known endocrine disruptor that affects various cells and tissues through estrogen receptors. BPA is also present in other polymer components, such as sterilizing filters, plastic bags, and tubing lines. There is a potential concern with polyurethanes used as potting compounds to secure hollow fiber membranes on both ends of a hemodialyzer that can lead to sensitization (specific IgE antibodies), asthma, or other side effects40.
THROUGH THE PORES: THE SEARCH FOR THE OPTIMAL FIT IN HEMODIALYSIS MEMBRANE DESIGN
Recent research has focused on developing more permeable membranes to achieve higher solute clearances. The expansion of the range of uremic toxins that can be effectively removed, specifically targeting middle to large-molecular-weight substances, is of particular interest.
High-cut-off (HCO) membranes are a type of HD membrane developed according to the rationale that a larger pore means better clearance of a broader MW molecule. By increasing the pore size, HCO membranes are pushing the boundaries of molecule clearance; consequently, potential risks of this pore size enlargement are expected. HCO membranes have pore sizes around 0.01 μm compared to high-flux membranes (pore sizes of 0.003-0.006 μm) or plasma-filtering membranes (pore sizes of around 0.2-1.0 μm); the pore sizes of HCO membranes are 2-3 times larger41.
HCO membranes have a cut-off permeability close to the glomerular basement membrane (65 kDa). HCO membranes, therefore, can clear large-middle molecules up to a MW ranging from 50 to 60 kDa that are elevated in blood during sepsis, such as cytokines (TNF-α: 17 kDa, IL-6: 26 kDa and IL-8: 8 kDa), rhabdomyolysis (myoglobin), and hematological disorders such as multiple myeloma (Free light Chains; FLC Kappa 22.2 kDa, and FLC lambda 45 kDa)42. Furthermore, PBUTs are frequently low MW solutes, but their binding to large serum proteins impairs their removal by dialysis and can be removed with HCO membranes.
Renal involvement is common in multiple myeloma, impacting about half of the diagnosed patients. The leading cause of kidney damage, known as cast nephropathy (myeloma kidney), is due to high blood levels of monoclonal FLCs. Early reduction in serum FLC concentrations in biopsy-proven myeloma kidney was associated with improved renal recovery and plasma exchange traditionally has been recommended to remove FLCs in myeloma kidney treatment43. The role of HCO HD for FLC in myeloma kidney was further investigated in two multicenter randomized trials that evaluated the efficacy and safety of HCO membranes for FLC removal in biopsy-proven myeloma cast nephropathy requiring HD. The MYRE trial44 was first published, randomizing 98 patients from 48 centers in France treated with bortezomib + dexamethasone to HCO-HD 5 h daily versus conventional high flux membrane (2.1 m2). There was no difference in dialysis independence at 3 months; however, a decrease in HD dependence at 6 and 12 months in HCO-HD was observed, with no difference in mortality. On the other hand, the EULITE trial45 randomized 90 patients from 16 centers treated with bortezomib + doxorubicin + dexamethasone to HCO-HD 6 h (first session) and 8 h thereafter versus dual membrane (1.1 m2). There was no difference in dialysis independence at 90 days; an increased risk of pneumonia at 90 days in HCO-HD was observed, with increased overall mortality in HCO-HD at 2 years.
HCO membranes have demonstrated the ability to improve hemodynamics and extend survival in experimental sepsis models46. Clinically, in patients with sepsis-induced acute kidney injury who underwent HCO-HD, a decrease in vasopressor requirements is observed as opposed to conventional CVVH with a significantly higher cytokine clearance47.
However, the limitation of these membranes is the protein loss, mainly albumin, due to the larger pore size. Excessive albumin loss could be a clinical issue and an important limitation of these treatments. Some studies found that using HCO membranes was associated with a large amount of albumin loss (up to 15 g per 4-h session)48 and even clinical hypoalbuminemia, which limited their routine application in chronic HD. HCO membranes can also lead to losing trace elements and other important molecules. To minimize albumin loss while maintaining cytokine clearance, it is recommended to use diffusion over convection49. Although the amount of albumin removal with HCO membranes is relatively small and could be supplemented, their use is indicated for only a few sessions in a diffusive mode for acute therapies.
Overall, HCO membranes represent an important advance in the field of HD, allowing for more efficient removal of large molecules and protein-bound toxins from the blood. However, their use may extend beyond these acute therapies to clear difficult-to-remove uremic toxins in selected chronic dialysis populations. Literature on HCO membranes exhibits significant heterogeneity because of variations in membrane type (cut-off points, surface area, and composition), modality (diffusive versus convective), and cytokine measurement. Therefore, definitive conclusions about the efficacy of this strategy are challenging. Ongoing research and development in this area will likely lead to further improvements in the safety and effectiveness of HCO membranes.
COMBINING KNOWLEDGE FOR BETTER DIALYSIS: THE ADVANTAGES OF MEDIUM CUT-OFF MEMBRANES
There is an acknowledgment that additional retention solutes beyond urea and low MW (MW range of 5KDa–50 KDa) have been linked to complications such as anemia, skeleton abnormalities, neuropathies, and dialysis-related amyloidosis, as well as unfavorable outcomes in dialysis patients. Consequently, current research is centered on developing therapeutic strategies to improve the removal of these substances. That was the rationale for developing HDF50. However, HDF requires specialized equipment, well-functioning vascular access, remarkably high blood flow, and large volumes of ultrapure dialysate and sterile substitution fluid; thus, it is unsuitable for all patients.
Recent advances in the membrane manufacturing industry have led to the development of a novel class of dialysis membrane called medium cut-off (MCO), which could better reduce the circulating levels of middle molecules (up to 45 KDa) beyond that of classic dialysis techniques, while allowing albumin to remain in the plasma51,52. The application of MCO membranes in a classic dialysis modality characterizes a new technique called expanded hemodialysis (HDx) to deliver expanded removal of middle and large molecular solutes that are typically retained by current dialysis therapy and to improve outcomes for maintenance HD patients53.
MCO membranes are composed of polyarylethersulfone (PAES/PVP) and have a mean pore radius of 5 nm and an effective pore radius of 3.0–3.5 nm after contact with blood54. The pore size is, therefore, suitable between high-flux and HCO membranes (Table 2). MCO membranes require the hollow fiber diameter to be reduced from the standard 200 µm to 180 µm, which increases the wall shear rate and blood flow velocity to avoid protein stagnation at the blood membrane interface and improve solute transport.
MCO membranes have a MW cut-off close to the MW of albumin and very high retention onset (HRO), previously called MCO and now defined as HRO51. MCO membranes exhibit a sieving curve demonstrating a progressive decrease in solute sieving as the solute MW of the solute increases53. The cut-off value of the membrane (MWCO) is defined by the MW of the solute, where 90% is retained (sieving = 0.1). Conversely, the retention onset of the membrane (MWRO) is defined by the MW at which 10% retention is observed (sieving = 0.9). The pore size distribution is critical in determining the different sieving properties. MCO membranes exhibit a size distribution that ranges from small to large, allowing for the removal of molecules of varying sizes. The pore sizes are strategically increased to optimize the removal of larger molecules without compromising albumin loss, although they remain smaller than albumin. In addition, MCO membranes have a tighter pore distribution than high-flux and HCO membranes to achieve a more uniform configuration. This tighter and more uniform pore distribution significantly enhances the permeability and selectivity of MCO membranes, enabling them to effectively filter a broader range of molecules while minimizing albumin loss. Figure 5 shows a representative pore size and pore size distribution across the membranes.

Figure 5. Representative pore size and pore size distribution across the membranes. Representative pore size and pore size distribution curves across different classes of membranes, low flux, high flux, medium cut-off (MCO), and high cut-off (HCO).
HDx combines diffusion and convection within a hollow fiber dialyzer with an MCO membrane. Convective transport is facilitated through a complex mechanism inside the dialyzer where filtration occurs in the proximal part, and backfiltration compensates for excessive filtration in the distal portion. An increased wall shear rate, due to the inner fiber diameter being reduced from the standard 200 µm to 180 µm, leads to improved solute transport by avoiding the secondary layer. The end-to-end pressure drop is also crucial in the internal filtration-backfiltration process. The effective internal filtration achieved in MCO membranes provides a remarkable convective clearance of medium to high MW solutes; therefore, a replacement solution is no longer required. The internal filtration (filtration-backfiltration) flow increases convective clearance and removal of middle and large MW substances55.
MCO membranes have been defined as HRO membranes due to the limited loss of albumin. The marginal loss of albumin observed in MCO membranes56 compared with HCO membranes is considered acceptable, if not beneficial, producing a certain clearance of protein-bound solutes.
This therapy does not need specific software or dedicated hardware, and a blood flow of 300 mL/min is sufficient to achieve optimal clearance, making HDx application possible in every setting where the quality of dialysis fluid meets current standards. Initial studies show that HDx provides clinical performances comparable with ol-HDF57. HDx may obtain additional benefits regarding clearances for solutes such as FLCs, whose clearance in HDF is marginal. A randomized study of 172 patients receiving either high-flux HD or HDx with Theranova® 400 showed that HDx provides superior removal of larger middle molecules (FLCs) while maintaining serum albumin level for 24 weeks58.
However, a study also reports a notable reduction in serum albumin, Vitamin D-binding protein, and a-1 acid glycoprotein59. Furthermore, long-term studies must explore other effects of HDx albumin loss and other biologically active proteins involved in hormones and drug binding.
ADVANCES IN SORBENT TECHNOLOGY
Adsorption is a highly efficient mass separation process that utilizes a specialized solid material called a sorbent to remove specific toxins from the blood9,10. Unlike traditional mechanisms of solute transport like dialysis, which rely on barriers, adsorption involves the interaction between the sorbent and the adsorbed molecules. This unique mechanism allows adsorption to overcome the limitations of dialysis membranes, enabling the effective removal of a broader range of solutes1.
The history of sorbents dates back almost two centuries and has been utilized for more than 50 years in blood purification treatments, beginning with inorganic aluminosilicates (zeolites) and charcoal, and followed by organic polymer ion exchange resins60. Today, biocompatible synthetic porous polymers based on materials such as styrene or acrylic acid have been utilized for blood purification61.
Sorbents are generally produced in granules, beads, fibers, cylindrical pellets, flakes, and powder62. They can be natural raw materials or be synthetically produced. Zeolites (alumina silicates) are inorganic polymers that possess natural porosity inherent in their structure. Porous carbons are organic polymers derived from cellulose through controlled thermal oxidation. Various reactions involving divinylbenzene as a potent cross-linking agent enable the synthesis of large molecules from almost any polymerizable monomer. These monomers can be bi-functional, forming linear polymers, or multifunctional, resulting in a cross-linked network polymer structure.
Sorbents are solid particles with a diameter generally ranging between 50 µm and 1.2 cm and are classified according to the size of the pores of their inner structure as: (a) Macro-porous (Pore size > 500 Å), (b) Mesoporous (Pore size 20–500 Å), and (c) Micro-porous (Pore size <20 Å)62. Sorbents possess a very large surface-to-volume ratio varying from 300 to 1200 m2/g61.
Sorbent materials must have high selectivity/affinity to bind specific solutes; this remarkable binding capacity is derived from its physical properties, including weak ionic bonds, Wan der Waals forces, and strong hydrophobic bonds.
A better understanding in recent years of the mechanisms involved in the adsorption process and the development of highly biocompatible new sorbent materials63 spread the application of adsorbent materials in various clinical settings requiring acute or chronic blood purification therapies known as HP or so-called hemoadsorption64,65.
In the blood purification technique of HP, blood is circulated by a pump through a sorbent bed or column (cartridge)66. During this process, solutes are removed from the blood by adsorption (binding) of molecules onto the sorbent particles. Sorbent materials such as activated charcoal, ion-exchange resins, polymyxin B, or other absorbent material are packed into this device (cartridge) that requires a tortuous pathway on which blood or any fluid phase are twisted, this allows for sufficient contact between the blood and the sorbent, enabling effective removal of target solutes65. An optimal packing design and density are required for an optimal mass separation process on the beads' surface. Maximum adsorption occurs at equilibrium, where the concentration of the marker solute at the outlet of the unit matches the concentration at the inlet62. HP can be integrated with other membrane-based modalities like HD to form HP-HD or CRRT to create HP-CRRT, allowing for the placement of the sorbent either before or after the dialyzer1,67.
In the past, HP techniques presented significant side effects and adverse reactions: chills, fever, cutaneous rash, thrombocytopenia, leucopenia, hypoglycemia, hypocalcemia, and aluminum leaching. These concerns delayed the application of HP in clinical practice. Adverse reactions induced by the interaction between blood and sorbent materials have become rare, primarily because sorbent materials are now designed to be bio or hemocompatible by a specific coating process covering the particles with bio-layers that are well tolerated by blood cells. Second, these reactions can be prevented by separating plasma from the blood before circulating through the sorbent bed (Plasmafiltration-adsorption). Following the sorbent cartridge, the blood components and plasma are recombined. This careful reconstitution process effectively prevents bio-incompatibility reactions from occurring68.
Potential side effects of HP include the unwanted removal of solutes such as antibiotics, anti-inflammatory substances, protective cytokines, or nutrients. However, in predominantly toxic conditions, the prevailing perspective suggests that the buildup of toxins is likely more significant than protective molecules. As a result, any removal method will eliminate a more substantial number of toxic molecules compared to protective molecules; nonetheless, unlike plasmapheresis or plasma filtration, which can non-selectively remove small solutes and proteins, including beneficial or necessary molecules such as clotting factors, albumin, antibiotics, and protective antibodies69. Consequently, replacing such losses requires the administration of albumin and fresh frozen plasma with the problem of cost and blood product consumption.
As a blood purification therapy, HP can remove a wide array of endogenous and exogenous toxins (including endotoxin, poisons, and drugs). Although there are no established indications for HP, several biological and pathophysiological rational indications have emerged.
End-stage kidney disease (ESKD)10 and acute kidney injury associated with sepsis and other critical illnesses represent possible areas of application of HP. The use of HP in poisoning or acute intoxications6,70, besides HD, is the treatment of choice in many instances due to the high affinity of the sorbent for the specific toxic molecule. HP includes the treatment of intoxication with drugs (e.g., valproate and carbamazepine) or poisonous chemical or natural products (e.g., organophosphates or mushroom-related toxins, respectively). HP devices, such as Cytosorb® cartridges and the Jafron® HA cartridges, have been used with 20-90% extraction rates. On ESKD patients, HP has been utilized as adjunctive therapy to HD to remove toxin solutes not adequately removed during dialysis. Recently, a prospective study71 of 400 chronic HD patients was randomized into four groups: low-flux HD, high-flux HD, HP + low-flux HD, or HP + high-flux HD. The HA 130 cartridge was utilized for HP groups. The study results showed that HP groups significantly improved b-2 microglobulin, PTH, and uremic pruritus score, irrespectively the HD membrane flow type.
In critically ill patients, sorbents are mainly applied for endotoxin and cytokine removal in conditions such as infection, intoxication, immune dysregulation, sepsis, and cytokine release syndrome. Endotoxin adsorption rationale has been based on trials studying the endotoxin-binding ability of polymyxin B (PMC) employing the Toraymyxin cartridge. The first one was EUPHAS72, a randomized study of 64 patients with septic shock due to abdominal cause by either PMX or conventional care. EUPHAS reported physiological advantages on blood pressure, gas exchange, and vasopressor use with PMX but no change in the control population. In addition, PMX decreased time to mortality. Conversely, a second randomized study73 of patients with septic shock due to peritonitis found no benefit and a trend toward earlier time to mortality with PMX versus conventional. The EUPHRATES74 trial compared PMX to conventional care in 450 critically ill patients with septic shock and an endotoxin assay activity of ≥ 0.60. Although this trial did not find a survival advantage, a post hoc assessment of patients without extreme endotoxemia found a survival advantage on time-to-event analysis.
Two new sorbent technologies have emerged: the Cytosorb75 cartridges and the Jafron® HA cartridges series. These sorbents have been used as rescue therapy in sepsis or as adjuvant therapy in sepsis, and they represent a form of generic (non-selective) anti-inflammatory strategy. Cytosorb® and Jafron® HA cartridges have been studied in case series and small comparative studies. In the past COVID-19 pandemic, Cytosorb® was tested with inconclusive results. Cytosorb® for > 72 h coupled to veno-venous extracorporeal membrane oxygenation (ECMO) in COVID-19 patients showed similar IL-6 levels compared with ECMO alone. However, after 20 days, the survival was 18 % with Cytosorb® and 76 % without (p = 0.0016); a potential Type 1 error has been suggested76. Later, a randomized controlled trial77 reported the effect of Cytosorb® therapy for 3-7 days in 50 COVID-19 patients with vasoplegic shock on time to resolution of shock. There was no significant difference in this outcome and a mortality of 78% with Cytosorb® compared with 73% in the control arm.
In addition to HP, there exists sorbent technology which exhibits technical aspects in relationship to HP with the fundamental distinction that, instead only the plasma made contact to the adsorptive cartridge, a process often referred to as plasma perfusion (plasma adsorption). This category encompasses the Plasmafiltration Adsorption (PFAD) and the Double Plasmafiltration Molecular Adsorption System (DPMAS)1.
Contrary to HP, plasma adsorption techniques achieve longer contact times due to the lower flow rates of plasma (typically ranging from 20 to 40 mL/min) as compared to the higher flow rates of blood (generally in the range of 150-200 mL/min). This extended contact period significantly enhances the efficiency of adsorption68,78.
In the case of PFAD, plasma is first separated from blood, circulated through the sorbent, and reinfused into the blood circuit. PFAD can be performed for a short period or over a prolonged period Continuous Plasmafiltration Adsorption (CPFA). Furthermore, like HP can be integrated with HD (PFAD-HD) or CRRT (CPFA-CRRT) to address specific clinical needs.
DPMAS, on the other hand, employs different (doubled) sorbent units with specific characteristics placed within the plasma circuit. The choice of the sorbent and the cartridge characteristics relay on the indications and target molecules79.
Importantly, plasma perfusion techniques possess the capability to remove a wide range of molecules, toxins, pro- and anti-inflammatory mediators, and cytokines in a non- selective manner. This unique attribute makes them a potentially valuable tool in the management of both acute and chronic liver diseases, among other clinical applications80,81.
A better understanding of the fundamental properties of each sorbent device and basic aspects of HP, PFAD, and DPMAS as an extracorporeal therapies: target molecules, their kinetics, indications, timing, number of sessions and duration of the treatment, outcomes to establish the efficiency/efficacy, and their financial implications will undoubtedly expand the potential for clinical application of sorbent devices82.
CONCLUSIONS
Advancements in HD membranes and sorbents have significantly improved the efficacy and safety of these blood purification therapies. The recognition of the detrimental impact of retention solutes beyond urea and low MW compounds on dialysis patients has spurred research into novel therapeutic strategies. These innovations have enabled removing a wider range of toxic solutes, particularly middle to large-molecular-weight substances associated with complications in dialysis patients, therefore improving patient clinical outcomes. However, to continuously improve outcomes, there is a need for ongoing research and innovation.
Adsorption stands a mass separation technique, utilizing specialized sorbent materials to efficiently eliminate specific toxins from the blood. The historical progression of sorbents, advancements on separation technique, and addressing biocompatibility issues has transformed HP and plasma adsorption into a promising therapy for conditions ranging from kidney diseases to critical ill patients. As this field evolves, refining sorbent properties, understanding their kinetics, and establishing optimal treatment protocols will further enhance their clinical potential for targeted toxin removal.