Introduction
Microbial mats from lagoons on the outer Pacific coast of the Baja California Peninsula (Mexico) have long been studied (i.e., Horodyski and Vonder Haar 1975, Stolz 1983, Spear et al. 2003, Omoregie et al. 2004). In contrast, lagoons along the inner shores of the peninsula, and on some of the many islands in the Gulf of California, were only recently found to harbor microbial mats (Johnson et al. 2012). In one of the earliest studies, Horodyski and Vonder Haar (1975) referred to their biological discovery at Laguna Mormona as stromatolites-the term applied by geologists and paleontologists to organosedimentary structures now regarded as microbial in origin. Stromatolitic fossils are now recognized as having a Pleistocene history in the Gulf of California (Backus and Johnson 2014), possibly dating back 712,000 y. In a recent overview of coastal ecosystems in the Gulf of California, 5 closed lagoons in the Bahía de Los Ángeles region were identified as habitats for active microbial mats (Johnson and Ledesma-Vázquez 2016). Other lagoons have since been explored, the most recent being Volcán Prieto Lagoon (so named herein), an isolated salt lagoon on the peninsular coast south of Puertecitos, first visited by one of the authors (JLV) in 2011.
Seventy-five kilometers south of the town of San Felipe, Mexican Highway 5 reaches the settlement of Puertecitos (Fig. 1). Three landmarks farther south of Puertecitos dominate the physical geography and related geology along this section of the Gulf of California shore (Fig. 1). Approached from the highway, the most distinctive feature, a cone-shaped structure called Volcán Prieto, sits 275 m above sea level. Mapped as a Pliocene volcano (Gastil et al. 1971), the basalt edifice is crowned by a crater roughly 350 m in diameter with a basin floor that sits from 8 to 10 m below the enclosing summit. Adjacent and to the north is a large fan delta that drains a network of streambeds belonging to Arroyo Los Heme (Fig. 2a). The delta takes its name from Playa Costilla at the far north end. At its widest, the delta is 3.75 km across but the front extends through an arc of 4.75 km. The longest tributary in Arroyo Los Heme follows inland through a winding course of 14 km entrenched in Pliocene volcanics defined as part of a large ignimbrite field (Stock 2000). At the highway crossing where the outer canyon opens onto the delta, the elevation is 50 m above sea level. From this low vantage, it is difficult to appraise the delta’s three-dimensional form, because the gradient through the central distributary channel to the sea amounts to only 2%. Incised distributary channels indicate that the delta has remained active, but it may have carried a greater sediment load in the past.
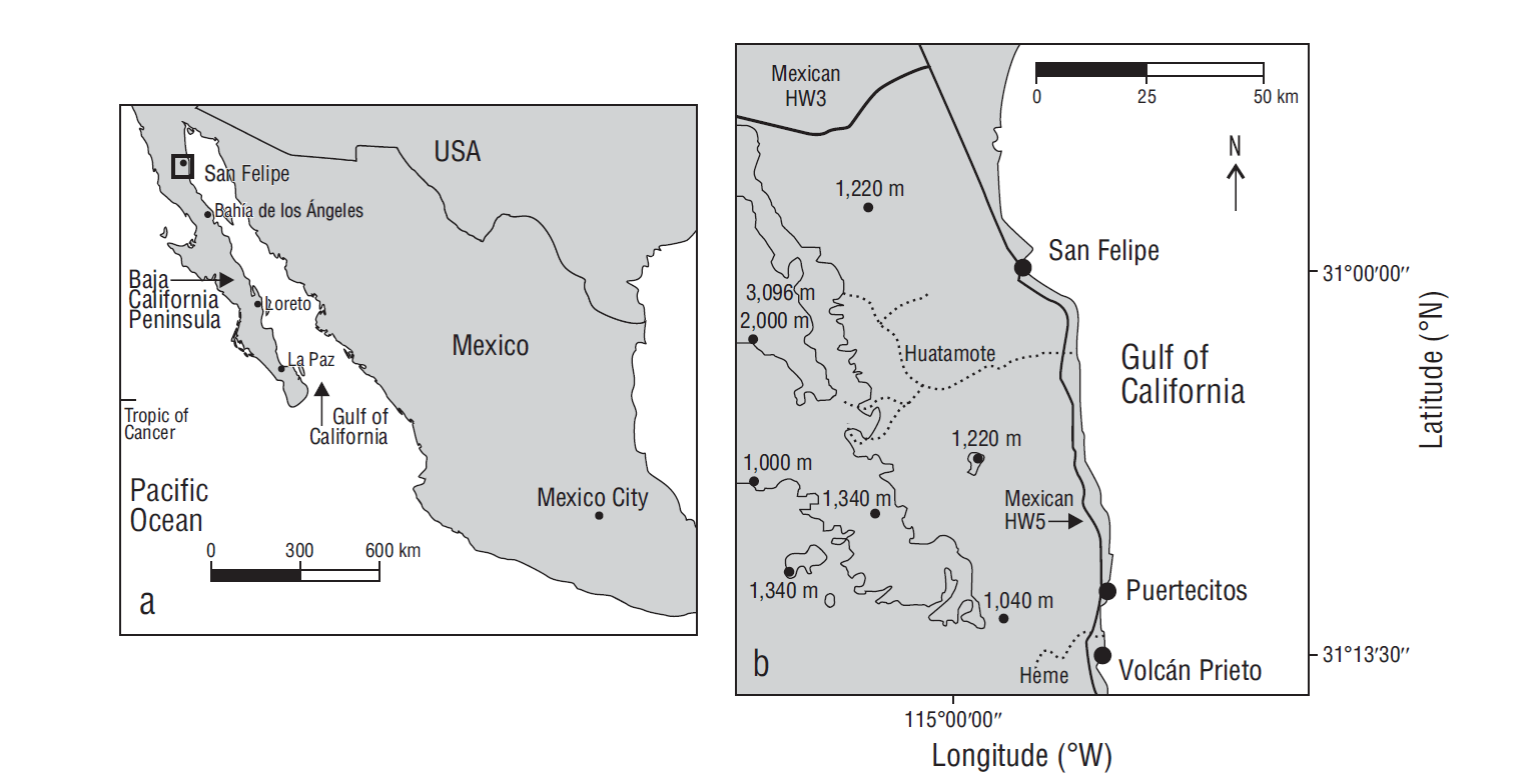
Figure 1 (a) Map showing the Gulf of Mexico and the Baja California Peninsula in relation to mainland Mexico; the box indicates the overall study region. (b) Map enlargement of the study region showing localities on the upper gulf coast of Baja California (HW stands for highway).

Figure 2 Photographs detailing the geomorphology around Volcán Prieto, south of Puertecitos, Baja California: (a) aerial photograph showing the prominent delta at the mouth of Arroyo Los Hemme, the cone and crater of Volcán Prieto, and the adjacent closed lagoon; (b) close view of the closed lagoon from the south flank of Volcán Prieto showing limestone (lmst) exposed in the lagoon and the site (X) where cores with microbialites were retrived; and (c) detailed view of the berm that isolates the lagoon from the open Gulf of California showing pumice cobbles (arrows) and small dark basalt boulders.
The closed lagoon at the south foot of Volcán Prieto is the area’s third landmark and the primary focus of this study (Fig. 2b). Crudely trapezoid in map outline, the lagoon front is defined by a coastal berm that fully separates it from the open Gulf of California. The lagoon’s maximum length in the landward direction is 750 m. A surface crust of white salt blankets much of the isolated lagoon (Fig. 2b). All 3 landmarks-the northern delta, the extinct volcano, and the southern lagoon-share essential thematic relationships in the area’s overall geomorphological history as related to patterns of local oceanic and atmospheric circulation in the Upper Gulf of California.
The Volcán Prieto Lagoon offers an excellent subject for complimentary studies on functional geomorphology and microbiology. The aims of this contribution are 2-fold: (1) to provide an overview of the physical geography of a closed lagoon and the stages through which it developed during post-Pliocene time, and (2) to offer a state-of-the-art case study on the rich biodiversity of microbial life, including both bacteria and archaea, in such an extreme habitat.
Materials and methods
Photography and site description
Perspective on the area’s key landmarks was gained during an overflight and from the aerial photographs that were taken. In the field, Volcán Prieto was climbed for the additional opportunities it provided for oblique views of Delta Costilla to the north and the Volcán Prieto Lagoon to the south. The lagoon’s berm was traversed for observations on its composition and construction. Other lagoon margins were examined for additional potential features regarding its history.
Core sampling
From the lagoon floor, 2 replicate cores were retrieved (14 May 2016) to obtain subsamples for molecular studies. Soft sediments were extracted using core barrels measuring 20 cm in length and 4 cm in diameter (Fig. 3). Gloves were worn during all stages of core retrieval and further treatment of subsamples. A metal spatula cleaned with 70% ethanol after each partition of the sample cores was used to introduce subsamples (each between 0.5 to 2.0 g) into 15-mL sterile plastic tubes containing RNAlater storage reagent (Sigma-Aldrich, Life Science) to preserve the nucleic acids. The ratio of RNAlater solution to sample was kept at 5:1. Vial caps were wrapped with parafilm to prevent contamination. Each core yielded a set of 4 subsamples collected at 5-cm intervals from the top of the core.
Genomic DNA extraction and quantification
Samples were washed in the laboratory (University of Vienna) with PBS buffer to remove RNAlater solution. A standard DNA extraction procedure including bead-beating and phenol-chloroform extraction with 5% CTAB/phosphate extraction buffer was used on washed samples (Gittel et al. 2014). Genomic DNA was quantified with a Qubit 2.0 Fluorometer (Life Technologies) before use in polymerase chain reaction (PCR) and amplicon sequencing.
Amplicon sequencing of 16S rRNA genes
For PCR amplification of small subunit ribosomal RNA (rRNA) genes, the primer pair U519F (5′ CAGCMGCCGCGGTAATWC 3′) and U805R (5′ GACTACHVGGGTATCTAATCC 3′) (V3-V4 region) was used in PCR to obtain gene fragments of approximately 300 bp. Library preparation and barcoding were performed, and high-throughput sequencing was done in-house on an Ion Torrent Personal Genome Machine using 300 bp chemistry and an Ion 316 v.2 Chip (Life Technologies). Sequencing data were quality filtered and trimmed with PRINSEQ allowing for a minimum and maximum read length of 220 bp and 310 bp, respectively. Quality of the sequences was then confirmed with FastQC v.0.11.5 before taxonomic assignment. Reads were clustered into operational taxonomic units (OTUs) with a 97% DNA sequence similarity cutoff and assigned using QIIME I (Caporaso et al. 2010) with the SILVA SSU Ref NR release 128 database as reference (Quast et al. 2013). QIIME I was also used to make rarefaction curves and principal coordinates analysis plots.
Lipid biomarkers
Four core subsamples (core 1) were dried in an oven at 40 ºC for 48 h, obtaining ~4 g of dry material for biomarker analysis. The samples were saponified with 6% potassium hydroxide in methanol and extracted 3 times by ultrasonication with dichloromethane:methanol (3:1). Total lipid extracts were pre-separated into n-hexane-soluble and dichloromethane- soluble fractions. The n-hexane-soluble fractions were further separated by column-chromatography into fractions of increasing polarity ( see Birgel et al. 2008). The alcohols were derivatized with BSTFA (N,O-bis- trimethylsilyl-trifluoracetamide) and pyridine (1:1) at 70 ºC for 1 h. The carboxylic acids were methylated with 20% boron trifluoride in methanol at 70 ºC for 1 h. Compounds were measured on a Thermo Electron Trace DSQ II gas chromatograph/mass spectrometer (GC/MS), and obtained mass spectra were compared with mass spectra from libraries and reference samples. The GC/MS was equipped with a 30 m HP-5 MS IU fused silica capillary column (0.25 mm internal diameter, 0.25 µm film thickness). The carrier gas was helium. The temperature program used for extraction of both fractions was as follows: 50 ºC for 3 min, raised from 50 to 230 ºC at 25 ºC per minute, and finally held for 3 min. Then, temperature was raised from 230 to 325 ºC at 6 ºC per minute. Finally, the temperature was held at 325 ºC for 15 min.
Results
Observations on local geomorphology
Aerial photography showed the proximity of Volcán Prieto on one side of the lagoon (Fig. 2a). The lagoon floor surface area was approximately 265,000 m2, 75% of which was covered by a crust of white salt. An oblique view of the lagoon from a closer range on the south flank of Volcán Prieto (Fig. 2b) showed a dark berm stretching straight across the lagoon front, unbroken for a distance of 530 m. A distinct patch with tan coloration formed a kind of island with low elevation behind the berm at the middle front of the lagoon. This feature turned out to be limestone (Fig. 2b) composed of fossil shells of the marine bivalve Chione californiensis (see Brusca 1980: p. 149, Figure 9.45 for representation of the extant shell).
With a cross-sectional width of 50 m, the berm was constructed from well-rounded basalt cobbles (Fig. 2c) that typically ranged between 10 and 25 cm in diameter. Less common, basalt boulders (from 30 to 50 cm across) also occurred on the berm. Pebble- to cobble-sized pieces of pumice occupied a long swath just below the berm crest parallel to the shore on the seaward side. These were orange in color and well-rounded with a maximum diameter of 12 cm (Fig. 2c). Samples from the pumice apron floated in seawater, and it can be assumed that the material settled on the berm during periods of high tide coinciding with higher than normal waves. The berm crest stood approximately 2 m above mean sea level. It is noteworthy that no pumice outcrops were observed during a traverse of Volcán Prieto.
Two cores were taken from the outer southern margin of the lagoon (Fig. 2b), where the overlying salt crust was relatively thin. The lagoon floor was soft and the weight of a person crossing the field was sufficient to break through the salt crust to dark, spongy material below. It was possible to penetrate the subsurface to a depth of 60 cm using the shaft of a shovel and when the probe was withdrawn, the resulting hole filled with water. This demonstrates that water saturation below the crust surface was quite high and in equilibrium with the level of the sea on the opposite side of the berm.
Maximum core length extruded from the core barrel was only 17 cm (Fig. 3). The top few millimeters of the core, directly below the salt crust exhibited a red pigment. Below, banded intervals occurred that showed a green pigment interspersed with colorless intervals. Within minutes after exposure to the air, subsamples cut from the core soon lost moisture giving the general appearance of uniform clay that concealed any former trace of organic pigmentation.
Abundance and taxonomy of Archaea and Bacteria
Analysis of 16S rRNA sequence data for relative abundances of bacteria and archaea throughout the replicate cores revealed that bacteria dominated all horizons, making up as much as 95% of total OTUs from the 10- and 15-cm horizons of both cores (Table 1). Main bacterial phyla included Proteobacteria, Planctomycetes, and Chloroflexi (Fig. 4). Within the Proteobacteria, the Gamma and Delta classes were most dominant (Fig. 5a). A wide diversity of less abundant bacteria were also observed in all horizons of both cores (Figs. 4, 5a).
Table 1 Total operational taxonomic units (OTUs) obtained from sequencing of core horizons, including relative abundance of Archaea and Bacteria within each horizon. Concentrations of genomic DNA (gDNA) per extraction also listed.
Core 1 | Core 2 | ||||||||
1 cm | 5 cm | 10 cm | 15 cm | 1 cm | 5 cm | 10 cm | 15 cm | ||
Total OTUs | 20,792 | 24,855 | 24,593 | 16,215 | 13,398 | 21,926 | 7,962 | 4,018 | |
Total Archaea OTUs | 2,423 | 7,272 | 4,441 | 898 | 1,408 | 6,446 | 424 | 210 | |
Archaea relative abundance (%) | 11.70 | 29.30 | 18.10 | 5.50 | 10.50 | 29.40 | 5.30 | 5.20 | |
Bacteria relative abundance (%) | 88.30 | 70.70 | 81.90 | 94.50 | 89.50 | 70.60 | 94.70 | 94.80 | |
gDNA (ng·L-1) | 2,620.10 | 318.50 | 74.50 | 33.60 | 1,285.00 | 297.90 | 16.30 | 27.50 | |
Sediment for extraction (g) | 0.50 | 0.63 | 0.68 | 0.69 | 0.58 | 0.77 | 0.48 | 0.84 |

Figure 4 Fluctuation in relative abundance of 16S rRNA from major archaeal and bacterial groups through horizons from core 1 and core 2. Bacterial groups PAUC34f, WS1, RBG-1, and BRC-1 represent taxonomic abbreviations for uncultured and/or candidate groups. For archaeal taxonomy, MEG stands for miscellaneous Euryarchaeota group and WSA2 for candidate methanogen class.

Figure 5 Relative abundance of 16S rRNA from major groups: (a) Bacteria and (b) Archaea. Unassigned sequences or sequences having <1% abundance are represented as “other”. Bacterial groups WS1, SBR1093, PAUC34f, RBG-1, and BRC-1 represent taxonomic abbreviations for uncultured and/or candidate groups. Abbreviations of archaeal phyla are as follows: Thaum, Thaumarchaeota; Wosese, Woesearchaeota; and Eury, Euryarchaeota. The Thaumarchaeota group is further identified as either soil Crenarchaeota group (SCG), marine group, or group C3 (uncultured archaeon).
When comparing OTUs per horizon, core 1 had a greater number of total OTUs per horizon than core 2, which coincided with less genomic DNA extracted from the respective horizons of core 2 (Table 1). Nevertheless, there were similar trends in the overall microbial community composition between the 2 cores (Figs. 4, 5). The relative abundance of archaea was highest in both cores at the 5-cm horizon (Table 1, Fig. 4), with Euryarchaeota dominating archaeal relative abundances. Euryarchaeota, particularly the class Halobacteria, made up between 40% and 75% of the archaeal abundance throughout both cores (Fig. 5b). Also, a diversity of newly identified archaeal phyla including Bathyarchaeota, Hadesarchaea, and Lokiarchaeota, only now starting to appear in studies due to increased sequencing efforts and in-depth phylogenetic analyses, appeared throughout the 2 cores (Fig. 5b). Rarefaction curves, created for the sequenced samples using QIIME, revealed that the sequencing depth was sufficient to capture phylogenetic diversity among both cores and with depth (data not shown). The principal coordinates analysis showed that the 1-cm layer was the most distinct in both cores and that when comparing the 2 cores, the samples clustered according to depth (data not shown).
GC-amenable lipid biomarkers
Characterization of the microbial biomass present in core 1 was done through analysis of lipid biomarkers. Lipid biomarker contents varied from 21 µg/g dry weight at 10 cm depth to a maximum of 537 µg/g dry weight at 1 cm depth (Fig. 6). The majority of lipids were found in the carboxylic acid fraction, making up 54 (10 cm) to 76 (15 cm) percentage by mass (wt%) of the total lipid inventory, followed by alcohols ranging from 23 to 46 wt%. Hydrocarbons were only detected in the uppermost layer (1 cm) with around 2 wt% and represented input from only cyanobacteria; at all other depths no biomarkers occurred in the hydrocarbon fraction (Table 2).
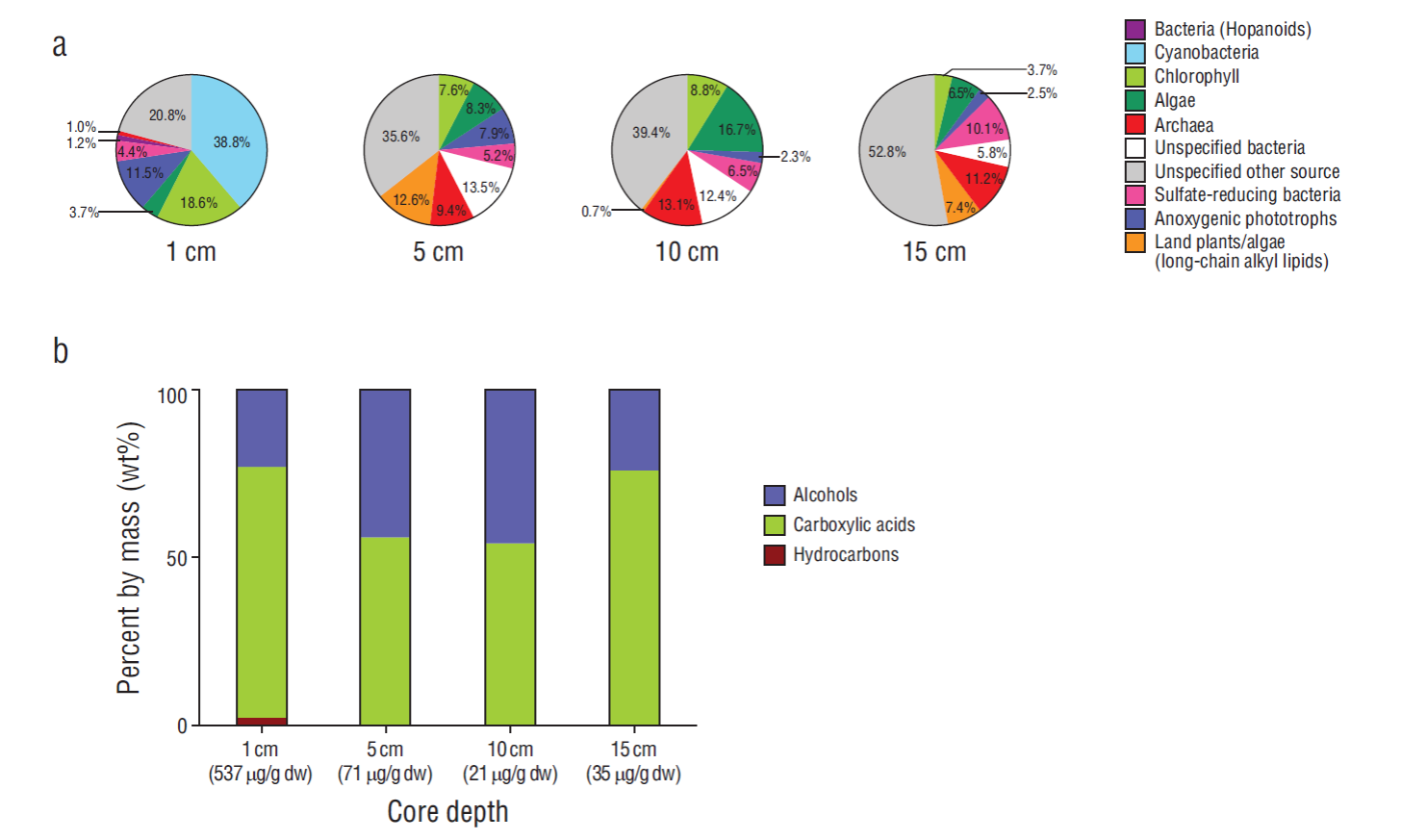
Figure 6 Lipid biomarkers assigned to source organisms and distribution of compound classes relative to depth. (a) Source-specific distribution of lipid biomarkers at 4 different depths in core 1 collected from the 3 fractions shown in (b). (b) Total amount (dw, dry weight) and percentage by mass (wt%) of all lipid compound classes from the 3 major fractions collected at 4 different depths in core 1. For a detailed list of biomarkers see Tables 2-4.
Table 2 List of hydrocarbon compounds, their sources, and their contents (dw: dry weight) at different depths in core 1.
Compound | source | 1 cm (mg/g dw) |
5 cm (Barren) |
10 cm (Barren) |
15 cm (Barren) |
n-C15 | Unspecified | 0.16 | - | - | - |
8-methyl-C16 | Cyanobacteria | 0.18 | - | - | - |
n-C17:1* | Cyanobacteria | 0.08 | - | - | - |
n-C17:1* | Cyanobacteria | 1.22 | - | - | - |
n-C17 | Cyanobacteria | 1.99 | - | - | - |
8-methyl-C17 | Cyanobacteria | 2.87 | - | - | - |
7-methyl-C17 | Cyanobacteria | Traces | - | - | - |
Phytene* | Chlorophyll | 0.87 | - | - | - |
n-C18 | Unspecified | 0.17 | - | - | - |
Phytene* | Chlorophyll | 1.55 | - | - | - |
Squalene | Chlorophyll | 3.12 | - | - | - |
Diploptene | Cyanobacteria | 0.48 | - | - | - |
Sum hydrocarbons | 12.68 | - | - | - |
*Position of double bond not assigned
The highest amount of source-specific lipids was found in the layer at 1 cm depth, where 79 wt% of all lipids were assigned to specific groups and the other 21 wt% represented by less specific lipids can be either derived from bacteria or eukaryotes (Fig. 6a). The major group of compounds were represented by cyanobacterial lipids (39 wt%, Table 2), followed by chlorophyll-derived lipids (both bacterial and algal- derived, 19 wt%) and lipids from anoxygenic phototrophic bacteria (12 wt%). Archaeal lipids made up only 1 wt% of all identified lipids. At 5 and 10 cm depth, lipid composition changed significantly, especially due to the lack of lipids specific for cyanobacteria (Fig. 6a, Tables 2-4). The sum of lipids assigned to different groups of organisms in the respective fractions ranged from 2 wt% (anoxygenic phototrophs at 10 cm) to 17 wt% (algae at 10 cm depth). Compared to the 1 cm depth sample, the variety of source organisms was greater below, and the contribution of lipids from unspecified sources (bacteria and eukaryotes) increased to 36 and 39 wt% at 5 cm and 10 cm, respectively. Archaeal lipids increased to 9 wt% at 5 cm depth and 13 wt% at 10 cm depth. At 15 cm depth, the amount of source-specific lipids decreased even more, and archaeal lipids represented 11 wt% of all lipids. Lipids from archaea and sulfate-reducing bacteria were the most abundant compounds (Tables 3, 4).
Table 3 List of alcohols, their sources, and their contents (dw: dry weight) at diff erent depths in core 1.
Compound | Source | 1 cm (mg/g dw) |
5 cm (mg/g dw) |
10 cm (mg/g dw) |
15 cm (mg/g dw) |
n-C14-alcohol | Unspecified | 1.94 | 0.18 | - | - |
n-C16:1-alcohol | Unspecified | 2.05 | - | - | - |
n-C16-alcohol | Unspecified | 1.54 | 0.53 | - | - |
br-C17-alcohol | Unspecified | 0.53 | 0.37 | - | - |
n-C17-alcohol | Unspecified | - | 0.21 | - | - |
Phytanol | Chlorophyll | - | 0.58 | - | - |
n-C18:1-alcohol | Unspecified | 2.97 | - | - | - |
n-C18-alcohol | Unspecified | 3.28 | 1.06 | 0.55 | 0.56 |
Phytol | Chlorophyll | 80.89 | 4.72 | 1.61 | 0.74 |
Tetrahydrogeranylgeraniol | Chlorophyll | 7.51 | - | - | - |
Geranylgeraniol | Chlorophyll | 1.81 | - | - | - |
n-C20-alcohol | Unspecified | - | 0.49 | - | - |
n-C22-alcohol | Unspecified | - | 1.16 | - | 0.88 |
n-C24-alcohol | Unspecified | - | 0.56 | - | - |
n-C26-alcohol | Unspecified | - | 1.24 | - | - |
n-C28-alcohol | Land plants | - | 1.87 | - | 1.04 |
Cholesterol | Algae | 2.35 | 0.81 | 0.45 | - |
Cholestanol | Algae | 1.92 | 0.87 | 0.40 | - |
Brassicasterol | Algae | 1.44 | 0.56 | - | - |
28Δ5-sterol | Algae | 4.20 | 0.89 | 0.74 | - |
29Δ5,22-sterol | Algae | 1.76 | 1.09 | 0.99 | - |
n-C30-alcohol | Land plants | - | 1.12 | - | - |
Sitosterol | Algae | 3.28 | 1.70 | 0.98 | 1.98 |
29Δ0-sterol | Others | - | 0.55 | - | - |
Tetrahymanol | Anoxygenic phototrophs | - | 0.94 | - | - |
Diplopterol | Unspecified bacteria | - | 1.11 | 1.18 | - |
Bishomohopanol | Unspecified bacteria | - | 1.79 | - | - |
Archaeol | Archaea | 5.14 | 5.30 | 2.83 | 3.36 |
Extended archaeol | Archaea | Traces | 1.36 | Traces | Traces |
Sum alcohols | 122.63 | 31.05 | 9.74 | 8.56 |
Table 4 List of compounds in the carboxylic acid fraction, their sources, and their contents (dw: dry weight) at different depths in core 1. FA = fatty acid, SRB = sulfate-reducing bacteria.
Compound | Source | 1 cm (mg/g dw) |
5 cm (mg/g dw) |
10 cm (mg/g dw) |
15 cm (mg/g dw) |
iso-C13-FA | Bacteria | - | - | - | 0.07 |
anteiso-C13-FA | Bacteria | - | - | - | 0.05 |
n-C13-FA | Unspecified | - | - | - | 0.09 |
iso-C14-FA | Bacteria | - | - | 0.08 | 0.16 |
n-C14-FA | Cyanobacteria (1 cm) | 1.87 | 0.42 | 0.34 | 0.76 |
iso-C15-FA | SRB | 7.10 | 0.56 | 0.31 | 0.50 |
anteiso-C15-FA | SRB | 2.56 | 0.45 | 0.24 | 0.40 |
n-C15-FA | Unspecific | 1.43 | 0.21 | 0.18 | 0.27 |
OH-C16-FA | Bacteria | 0.79 | 0.17 | 0.08 | |
iso-C16-FA | Bacteria | 4.68 | 0.73 | 0.29 | 0.60 |
n-C16:1-FA | Cyanobacteria (1 cm) | 2.95 | 0.31 | 0.23 | - |
n-C16:1-FA | Cyanobacteria (1 cm) | 21.39 | - | - | 0.42 |
n-C16-FA | Cyanobacteria (1 cm) | 174.80 | 5.40 | 2.20 | 5.02 |
10Me-C16-FA | SRB | 6.65 | 0.96 | 0.38 | 1.17 |
br-C16-FA | Bacteria | 1.02 | 0.37 | 0.14 | 0.16 |
iso-C17-FA | SRB | 2.51 | 0.61 | 0.15 | 0.35 |
anteiso-C17-FA | SRB | 2.14 | 0.75 | 0.19 | 0.45 |
n-C17:1-FA | SRB | 2.71 | 0.38 | 0.11 | 0.21 |
n-C17-FA | Others | 3.84 | 0.50 | 0.16 | 0.31 |
iso-C18-FA | Bacteria | - | 0.40 | 0.14 | 0.17 |
Phytanoic acid | Chlorophyll | 4.89 | 0.68 | 0.26 | 0.37 |
n-C18:1-FA | Unspecified | 53.38 | 1.53 | 0.77 | 0.83 |
n-C18:1-FA | Unspecified | 25.20 | 0.56 | - | 0.26 |
n-C18-FA | Unspecified | 20.33 | 3.09 | 1.37 | 2.32 |
CycC19-FA | Anoxygenic Phototrophs | 61.60 | 4.67 | 0.49 | 0.77 |
n-C20-FA | Unspecific | - | 1.20 | 0.29 | 0.46 |
n-C21-FA | Unspecific | - | 0.34 | - | 0.17 |
n-C22:1-FA | Unspecific | - | 0.78 | 1.14 | 0.99 |
n-C22-FA | Unspecific | - | 1.32 | 0.31 | 0.76 |
n-C23-FA | Unspecific | - | 0.43 | - | 0.29 |
n-C24-FA | Unspecific | - | 2.38 | 0.88 | 1.44 |
n-C25-FA | Unspecific | - | 0.54 | - | 0.25 |
n-C26-FA | Land plants | - | 1.67 | 0.15 | 0.63 |
n-C27-FA | Land plants | - | 0.52 | - | 0.15 |
n-C28-FA | Land plants | - | 1.74 | - | 0.44 |
n-C29-FA | Land plants | - | 0.22 | - | |
n-C30-FA | Land plants | - | 0.58 | - | - |
17α(H),21β(H)-32-hop-17(21)-enoic acid | Bacteria | - | 0.59 | - | - |
17 α(H),21β(H)-32-hopanoic acid | Bacteria | - | 0.95 | - | - |
17 β(H),21 β(H)-32-hopanoic acid | Bacteria | - | 3.54 | 0.82 | 0.49 |
Sum carboxylic acids | 401.84 | 39.57 | 11.63 | 26.87 |
Overall, bacteria and eukaryotes were the main sources of the majority of lipids in the studied core. Archaeal lipids measured on the GC/MS included only diether lipids, which are especially prominent in Euryarchaeota. Two diether lipids, archaeol and extended archaeol, were present (Table 3). Whereas archaeol was present at all depths, especially from 5 to 15 cm depth, extended archaeol was only detected at 5 cm depth, and in traces at other depths. Extended archaeol is a characteristic biomarker for halophilic euryarchaea (e.g., Dawson et al. 2012). We searched for the second prominent group of archaeal membrane lipids, glycerol dibiphytanyl glycerol tetraethers (GDGTs), using a high-performance liquid chromatography/mass spectrometry system; however, the contents of GDGTs were too low to be detected.
Discussion
Significance of local geomorphology
Arid lands attributed to the San Felipe Desert Subregion on the Upper Gulf of California constitute a southwestern extension of the Sonoran Desert. Normally, this area is the driest part of Baja California with an average annual rainfall of 5 cm (Rebman and Roberts 2012). During the winter months (November through March), strong winds generated by passing high-pressure systems north of the USA-Mexico border may detach and funnel through the axis of the gulf from north to south for several days at a time. These winds are responsible for vigorous longshore currents that scour peninsular shores on the gulf coast, moving beach sediments southward and even affecting the disposition of coastal dunes beyond La Paz in the far south (Johnson and Ledesma-Vázquez 2016). During the summer months (May through September), the wind field over the Gulf of California is prone to reverse itself, often sending lighter, moisture-laden winds northward.
Especially during late summer and early fall, tropical storms that originate off the Mexican mainland coast well south of the Baja California Peninsula intensify in strength and send hurricanes out into the Pacific Ocean (Romero-Vadillo et al. 2007). Every 6 to 8 y during El Niño events, some storms make their way northward into the Gulf of California. The last significant rain storms to affect the San Felipe Desert Subregion occurred in 2003 and 2014 under the influence of hurricanes Marty and Odile, respectively (Johnson and Ledesma-Vázquez 2016). Although downgraded to subtropical storms after initial landfall at the southern end of the peninsula near the Tropic of Cancer (Fig. 1), these events brought sudden deluges of rainwater to a climatic zone characterized as a “dry domain” normally controlled by dominant high-pressure systems around 30ºN (Bailey 1998).
Local evidence of heavy rainfall is apparent across the area under study in this project. Notably, the Volcán Prieto crater, 350 m in diameter, is floored by silt that shows up in aerial photography as a pair of white patches (Fig. 2a). Exploration of the volcano during fieldwork confirmed that the material on the crater floor derived from a restricted source area on the surrounding crater walls. The only basis for this deposit comes from repeated exposure over time to storm cells that sat above Volcán Prieto for even a few hours at a time.
Delta Costilla immediately north of Volcán Prieto also attests to repeated flood events draining Arroyo Los Heme over time, also resulting in the deep entrenchment of streambeds in the region’s greater Pliocene volcanoclastic terrain. Given the lack of pumice on the slopes of Volcán Prieto, it is likely that the pumice cobbles found on the lagoon berm to the south originated inland and were carried to the Gulf of California through Delta Costilla. Once in gulf waters, longshore currents were responsible for transporting the pumice cobbles to the lagoon berm. Essentially, these distinctive cobbles stranded on the lagoon berm are the equivalent of bathtub “yellow duckies” that float around and trace water movements. The more substantial basalt cobbles and boulders on the berm must have their source from the eroded gulf shores of Volcán Prieto.
Paleontological aspects of the Volcán Prieto Lagoon indicate that the place was formerly an embayment with an open connection to the Gulf of California. In particular, the limestone “island” behind the berm at the middle front of the lagoon has abundant fossils dominated by the marine bivalve C. californiensis. The deposit most likely dates from the last interglacial episode during the late Pleistocene roughly 125,000 y ago. The same species survives in the Gulf of California, today, and occupies a habitat limited to the “low tide mark, on mud flats, and offshore” (Brusca 1980, p. 146).
In summary, the post-Pliocene history of the Volcán Prieto Lagoon tells a story starting with an open embayment occupied by marine invertebrates living in an intertidal to shallow subtidal setting as recently as 125,000 y ago. Under wave attack, coastal erosion of adjacent Volcán Prieto led to the transport of basalt cobbles and boulders to construct a spit that gradually extended in length to close off the Pleistocene embayment. With isolation from open waters in the Gulf of California, the closed lagoon was subject to long periods of aridity interspersed with short spurts of rainfall.
The region’s more recent record of episodic rainfall clearly has had an effect on the Volcán Prieto Lagoon. Shallow pools of standing water were observed in the lagoon, when one of the authors (JLV) first visited in 2011. This has possible ramifications for the lagoon’s biology, which would need to withstand extreme fluctuations between freshwater immersion and long periods of extreme aridity when the salinity of the lagoon water turns briny. It is likely that the standing water from 2011 was left over from rainfall dropped during the passage of Tropical Depression Georgette, which crossed the region in September 2010.
Significance of local microbial biology
Previous research by Johnson et al. (2012) on the microbial biology of closed lagoons located farther south of the Puertecitos district in the Bahía de Los Angeles region, in the Gulf of California, identified only a few microbial genera. These were found to include filamentous cyanobacteria such as Phormidium, Oscillatoria, Geitlerinema, and Chroococus together with the solitary cyanobacterium Chroococcidiopis. Older research on lagoons from the Pacific shores of Baja California (Horodyski and Vonder Haar 1975, Horodyski 1977) emphasized even fewer microbial genera dominated by filamentous cyanobacteria such as Microcoleus and Lyngbya together with the solitary cyanobacterium Entophysalis. The current work, which is based on high throughput sequencing of 16S rRNA gene fragments, allowed deeper insights into the microbial diversity than before and revealed a greater number of taxa (OTUs) based on a 97% similarity exclusion criterion. It also revealed an impressive diversity on higher levels (i.e., order, family, and phyla) for Bacteria and Archaea. Nevertheless, in the surficial sample from 1 cm depth, lipid biomarkers indicate strong dominance of cyanobacteria, with their lipids representing approximately 40 wt% of the total lipid inventory. The lipids assigned to cyanobacteria comprise fatty acids with 14 and 16 carbons, but also the highly- specific normal, unsaturated, and methyl-branched heptadecanes and heptadecenes (e.g., Gelpi et al. 1970, Thiel et al. 1997). The latter lipids are exclusively produced by various cyanobacteria.
At least 8 major archaeal phyla and 25 major bacterial phyla were dominant throughout both cores according to amplicon sequencing results (Fig. 4). The majority of archaeal representatives in both cores were largely from newly identified and/or candidate phyla lacking cultured representatives (Hug et al. 2016). In particular, these include the Woesearchaeota and members of the DPANN superphylum (Castelle et al. 2015) (Fig. 5b). Most importantly, the current study supports the potential for a halophilic lifestyle for these archaea. There is a significant possibility that the sediment, at least in the uppermost layers, is highly saline given the presence of a salt crust flooring the lagoon. The salt crust is likely formed through seawater passing throughout the sediment layers of the lagoon and being driven upward by intense evaporation caused by high temperatures in the region. Furthermore, this implies that seawater remaining in the sediment could be highly saturated. Euryarchaeota are common inhabitants of high-saline ecosystems (Andrei et al. 2012), particularly the Halobacteria. Obligate halophiles can withstand salinities 10 times that of seawater (Ng et al. 2000). Not unexpectedly, Halobacteria were found throughout all horizons of both cores (Fig. 5b). A clear stratification was seen for 2 groups, the Thermoplasmata, mostly confined to the upper 1 cm of both cores, and the Thaumarchaeota, mostly occurred in the deeper layers (10 and 15 cm, Fig. 5b). The latter group is found commonly and in large amounts in ocean waters and marine sediments and represents chemolithoautotrophic organisms involved in the first step of nitrification. That is, they are known to gain energy from the oxidation of ammonia to nitrite (Schleper and Nicol 2010). Interestingly, this group is not usually associated with hypersaline environments. Thermoplasmatales, particularly marine benthic group D (MBG-D), were found to dominate clone libraries of artificial hypersaline ponds in Guerrero Negro, Baja California Sur, Mexico (Jahnke et al. 2008). The MBG-D were of highest abundance among Thermoplasmatales phylotypes in the present study when looking more in depth at archaeal diversity (data not shown). Regarding archaeal biomarkers, the diether membrane lipids archaeol and extended archaeol were identified in high amounts, representing input from apparently dominant halophilic Euryarchaeota (Dawson et al. 2012). Especially at 5 cm depth, extended archaeol, a highly specific biomarker for halophilic Euryarchaeota, was very abundant. The other archaea identified by high throughput sequencing of 16S rRNA gene fragments could not be recognized by lipid biomarkers, probably due to too low contents or the lack of specific lipids. However, since the lipid biomarker inventories of the newly identified and/or candidate phyla lacking cultured representatives are not known to date, future work may allow for the detection of these phyla with biomarkers.
Bacteria made up the significant portion of OTUs, from 70% to 95%, in both cores at all horizons (Table 1). Although Planctomycetes and Chloroflexi were dominant bacterial phyla in both cores (Fig. 4), a great diversity of bacterial phyla was recognized in all core horizons (Fig. 5a) in agreement with previous findings on the hypersaline microbial mats of Guerro Negro (Ley et al. 2006). Stratification among the different horizons at the Volcán Prieto Lagoon was less pronounced for archaea in comparison to bacteria; however, more replicate cores would be needed to confirm such statement. Comparable studies of bacterial diversity in certain saline ecosystems that also used high throughput sequencing have found similar phyla as the current study (Canfora et al. 2014), but also suggest a need for further research on the topic as many of the phyla not normally associated with high salinity are among the dominant community members. We assume that the large diversity in this extreme arid setting is favored by the fluctuations in salinity, which in turn favors the propagation of microorganisms with different adaptations (from medium to high salinity) either at different times or in different layers of the growing biofilm. It is also likely that a fluctuation in nutrient input throughout the lagoon sediment, particularly as seawater is driven upward, would cause stratification of microorganisms based on metabolic adaptation to available electron donors and acceptors. Important analyses on sediments of the lagoon that go beyond taxonomic affiliation are still needed to elucidate the active microbial communities. These should include metagenomic and metatranscriptomic approaches. These approaches could (1) provide new genomes of phyla for which there are currently no, or very few, sequenced representatives; (2) highlight functional redundancies within the communities as well as unique niches; (3) showcase metabolic diversity of bacteria and archaea found in saline environments; and (4) provide a further marker for active and non-active members within the community.
The lipid biomarker inventories revealed significant changes with depth (Fig. 6). At 1 cm depth cyanobacteria predominated, indicated by the high abundance of heptadecanes (Gelpi et al. 1970, Thiel et al. 1997), and the only other group that contributed significantly to biomass production were anoxygenic phototrophic bacteria, indicated by the presence of a cycC19:0 fatty acid (e.g.,Grimalt et al. 1992, Bühring et al. 2009). At 5 cm depth cyanobacteria- specific lipids disappeared, but chlorophyll and algal markers remained present with around 16 wt%. Anoxygenic phototrophic bacteria decreased (8 wt%) at this depth, but other unknown bacteria increased to 14 wt%; some of the lipids that cannot be assigned to specific bacterial lineages include hopanoids. However, the terminally branched fatty acids could be assigned to sulfate-reducing bacteria (e.g., Heindel et al. 2012), representing 5 wt% of all lipids at 5 cm depth. Whereas anoxygenic phototrophic bacteria became less abundant with depth, sulfate-reducing bacteria slightly increased with depth.
The area south of Puertecitos in the San Felipe Desert Subregion along the Upper Gulf of California is special for its rich physical landmarks and the high level of microbial biodiversity discovered in natural salt lagoons. The closed lagoon studied here retains sufficient physical evidence to reconstruct its origins from an open embayment with normal marine circulation on the sheltered side of a Pliocene volcano. Over time, extension of a spit composed of coarse clastic materials eroded from the extinct volcano’s shoreline closed off the embayment to create an isolated lagoon. Recent history of the Volcán Prieto Lagoon suggests that prolonged periods of extreme aridity are punctuated by brief episodes of intense rainfall lasting perhaps only hours. Such a lagoon setting with wide variations in standing floodwater reverting to salt brine provides a refuge habitat distinctly suitable for microbial life.
Our study indicates that a great diversity of microorganisms of well-known and also recently discovered, but currently uncharacterized, bacterial and archaeal phyla thrive in natural lagoons. It is of great interest for future studies to distinguish actively growing cells from dormant or dead material that accumulates as the biofilms accumulate in this fluctuating environment. In addition, further studies are needed to confirm the present finding with respect to temporal changes in community composition, as well as analyzing other similar settings in different regions of the Gulf. This will allow for statistical analyses of microbial community compositions in the context of different geographical and environmental parameters through a north-south climatic moisture gradient to understand which factors drive diversity.