Introduction
We had the great pleasure of writing the first comprehensive book on biological corridors, Corridor Ecology, with William Z. Lidicker in 2006 (Hilty et al. 2006) and significantly updating it together in 2019 (Hilty et al. 2019). Here we define a corridor as any space that facilitates the movement of populations, individuals, gametes or propagules, and plant parts capable of vegetative reproduction, in a matter of minutes, hours, or over multiple generations of a species. During the earliest meetings, Bill Lidicker urged us to specify who the corridors are for. What are the focal species? After all, species have different habitat preferences, and many species use one habitat type for part of their life cycle and a different habitat type for another. For example, amphibians may require wetlands or rivers during early life stages, upland habitat during the adult stage, and suitable substrate between the two for safe passage (Ribeiro et al. 2011).
The introduction of landscape ecology into the study of demography added many new insights for ecologists and conservation biology (e. g.,Hanski 1999; Turner 2005). Lidicker’s work led to an increased appreciation that the spatially explicit mosaic of habitat patches, edge effects, corridors, and even the proportion of favorable to marginal habitats can all be critically important factors in influencing population dynamics (Lidicker 2002). Hence, habitat-patch configuration and connectivity influences effective population size, density dependence, and growth and decline of species (e. g.,Cushman 2006; Lidicker 1994). Clearly, determining if and how habitat patches should be connected is important to prevent species decline. This argues for focal-species based approaches that, if taking climate change into account, rely on modeling current and future species distributions and movements to identify corridors that have the potential to facilitate movement, or help species move to regions that will become suitable for them (e. g.,Dilts et al. 2016; Wasserman et al. 2013).
We (Jodi and Adina) often argued against the importantance of desiging corridors for specific focal species and suggested a need to shift from planning habitat connectivity for a few well-known focal species to an ecosystem approach where the objective is for the entire biota to move through the landscape (Lawler et al. 2015). For example, by identifying and designing structural corridors that take advantage of readily available spatially explicit land-cover data, conservation biologists can delineate open space presumed to be permeable from the built environment (Theobald et al. 2012). Admittedly, it can seem premature to suggest methods for enhancing connectivity when not enough is known about the requirements of the species presumed to need it. However, in-depth understanding of species habitat and dispersal requirements is difficult to obtain.
Despite this and other debates on the topic, there was a need for a general text on habitat corridors and together we wrote the first book about habitat connectivity science and practice that included structural and focal species approaches to corridor design (Hilty et al. 2006). This essay provides a critical assessment of commonly used methods, and where designated corridors fall short for species most in need of connectivity conservation. In essence, we circle back to Lidicker’s point about the importance of considering species ecology and population viability.
Structural connectivity designs that meet the needs of many species or whole ecosystems are based on the level of human impact or naturalness and sometimes use graph theory to prioritize connections between habitat patches (Kong et al. 2010; Minor and Urban 2008). However, this approach often lacks information on animal ecology. This can lead to corridors that may not work for species with special needs. Hence, fine-filter approaches that are informed by species ecology may be necessary particularly for individual species that slip through the coarse filter employed by structural connectivity measures and may require specific conservation action particularly in the face of climate change (Hunter 2005).
Here, we describe the role of ecological connectivity as a global biodiversity strategy and review widely used structural or coarse-filter approaches, and where they may fall short for species most in need of connectivity conservation. We draw on meta-analyses that reveal species traits which may increase extinction risk and reduce resilience to climate change. Finally, we suggest research directions to fill gaps between structural-connectivity analyses and conservation planning for the most vulnerable species, with a focus on dispersal and movement biology and ecological monitoring. Improved approaches to connectivity modeling and field studies may provide a way forward to help conserve those species that most need it in a rapidly changing world.
Why habitat connectivity? Global estimates of terrestrial and marine species-extinction and population-extinction rates are several orders of magnitude higher than background levels (Ceballos et al. 2015; Payne et al. 2016) and continue to accelerate (Isbell et al. 2017). With even some abundant species on the decline, many local populations going extinct, and massive shifts in ecosystem composition expected due to climate change, it is no surprise there is a strong call for increasing the number and size of protected areas (Ceballos et al. 2017; Hallmann et al. 2017; Urban 2015). Clearly, ensuring the persistence of biodiversity requires effective protection of a percentage of the Earth’s surface, although just how much is debated (Woodley et al. 2019). Ecological connectivity is the second key component for biodiversity conservation, because protected areas become increasingly isolated due to surrounding land degradation, and the resident populations become more vulnerable to random genetic and demographic changes, increasing the risk of extinction (DeFries et al. 2005; Fagan and Holmes 2006). Even the largest protected areas will lose species due to isolation and external environmental pressures over the long term (Halley et al. 2016; Laurance et al. 2012).
In addition, even large protected areas may not be climatically diverse enough to retain their set of species with rising temperatures. In fact, with climate change no country is expected to retain the current level of protection for even half of the range of current climatic conditions found in their existing areas (Elsen et al. 2020). Therefore, ecological connectivity is one of the top recommendations for building ecosystem resilience around climate change, and is often the only option when surrounding landscapes are heavily modified (Heller and Zavaleta 2009). The importance of connectivity in addition to protection was recognized in the 2010 version of the Convention on Biological Diversity, where the Aichi Target 11 was set to have 17 % of Earth’s land surface covered by well-connected PA systems by 2020. There is also increasing recognition of the importance of “climate-wise connectivity”, which aims to connect current habitat to future suitable habitat, not just by facilitating daily, dispersal, or migratory movements, but over the generations during which range shifts are likely to occur (Keeley et al. 2018).
Many large-scale conservation efforts are actively seeking to identify, restore, and create protected areas and corridors as part of large landscape and seascape initiatives. Examples are Australia’s Great Eastern Ranges corridor; Two Countries One Forest in North America’s Eastern Appalachian region; Europe’s Natura 2000 conservation network; the Yellowstone to Yukon Conservation Initiative; Baja California to Bering Sea in the Pacific Ocean; and the Vatui-Ra Seascape in Fiji (Hilty et al. 2012). With ecological connectivity central to the implementation of regional, national, and international conservation efforts, the IUCN produced Guidelines for Conserving Connectivity through Ecological Networks and Corridors to provide guidance for governments and conservation practitioners to plan and implement ecological networks consisting of protected areas and corridors (Hilty et al. 2020).
Structural connectivity. Designing structural connectivity as a coarse-filter approach that accommodates the need of many species is often recommended as the first step for designing corridors (Beier et al. 2011). Here we use the term ‘structural connectivity’ to refer to methods that rely mostly on land cover information and patch configuration with little or no information on species behavior or habitat preferences. In developed and agricultural areas, remnant vegetation identified as structural connectivity often are de facto corridors, such as fencerows, windbreaks, roadside vegetation, and creeks or ditches that serve as structural elements that may function as corridors (Bennett 1990; Kasten et al. 2016; Kubeš 1996). They may harbor optimal habitat or, more commonly, marginal habitat left undisturbed as development progressed, providing vegetative structure that is distinct from the surrounding developed matrix.
Planners attempt to locate corridors to go through areas presumed to offer the least resistance to movement therefore are least costly to move through. Landscape resistance estimates are often at the heart of identifying corridors. Resistance estimates are generally expressed as a spatial raster layer made up of grids of cells where each cell contains a value representing information that represent the hypothesized relationships between landscape features (e. g., land cover, topography) and the ability for organisms to move and influence gene flow (Spear et al. 2010).
Several studies use the degree of human modification or level of naturalness to estimate resistance. This approach assumes, for example, that species avoid highly developed areas such as urban or intensive agricultural land cover (Gray et al. 2016). Some studies also add elevation, slope, and large rivers into the resistance calculation to account for the tendency of species to avoid steep terrain and crossing large rivers (Dickson et al. 2017). Another structural approach aims to find corridors consisting of similar land facets as present in the natural areas to be connected. Land facets are landscape units defined bytopography and soil type (Brost and Beier 2012). This approach is based on the assumption that species are often adapted to particular types of land facets, for example, steep, north-facing slopes, and will preferentially move between protected areas through corridors that reflect this type of physiography. The level of dissimilarity of a landscape cell to the focal facet type is used as a measure of resistance. If the resistance estimates used are inaccurate, the identified corridors may not function as intended. In fact, when a combination of focal species models was compared with structural approaches to identifying connectivity areas, the structural model had greater spatial overlap with corridor networks designed for far-dispersing large-bodied species than smaller-bodied taxa (Krosby et al. 2015).
Survey data collected by Keeley et al. (2019) from authors of 109 connectivity conservation plans reveals that a majority (79 %) were designed with focal species in mind (Figure 1). Thirty percent of the plans took advantage of one or more species-explicit modeling approaches, 13 % used individually based movement models, 9 % used meta-population models, and 17 % used species distribution models (24 % of plans applied two or all three approaches). Most of the other plans (39 %) incorporated information about the focal species to help estimate the resistance of the landscape to species movement or relied in part on local knowledge or expert opinion around species preferences and movement patterns. Climate gradient information was rarely incorporated (4 %) into the data used to develop these plans. This shows that while some species information is often used when creating connectivity-conservation plans, extensive data requirements on the ecology of focal species are a challenge for developing fully informed species-based approaches.
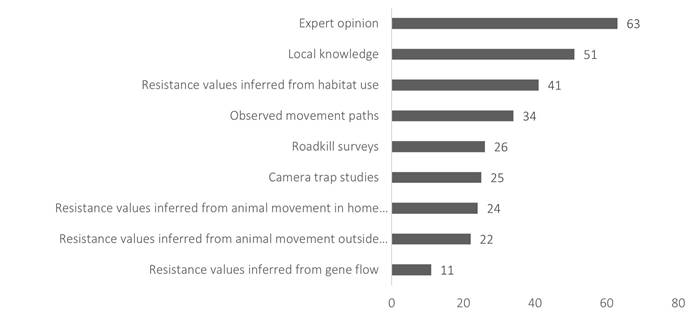
Figure 1 Data sources informing connectivity modeling in 109 connectivity conservation plans; see (Keeley et al. 2019) for data collection methods. One plan may use more than one data source.
Structural climate-wise connectivity. Climate change provides an additional motivation for establishing large-scale ecological networks, because such networks would allow for species range shifts (Lawler et al. 2015). At the same time, there is concern that areas established to protect particular species will, due to climate change, not be suitable anymore for these species. Instead, the need to protect them will arise in other places that become climate refugia (Alagador et al. 2014). Several connectivity-modeling approaches designed to be climate-wise take into account estimates of climate velocity, climate analogs, climate gradients, and climate refugia, combined with structural-connectivity approaches such as resistance estimates based on low human impact.
Today’s protected areas can be connected to sites that are forecasted to harbor analogous climate characteristics in the future. Ensuring habitat connectivity between climate analogs does not make any assumption about the effect of climate change on species responses. Therefore, increasing connectivity between climate-analog sites can be a useful strategy for climate-wise structural-connectivity designs intended to facilitate movement for entire communities (Littlefield et al. 2017; McGuire et al. 2016). Similarly, climate-gradient corridors connect climate-analogue natural areas with corridors that harbor similar temperature and precipitation regimes, avoiding steep climatic gradients along the corridor (Nuñez et al. 2013). Forecasting local climate change is inherently uncertain and this uncertainty should be explicitly incorporated when employing climate analogs and other methods that rely on climate models (Mozelewski and Scheller 2021).
Measures of climate velocity, calculated as the rate of change per time divided by the spatial gradient of change (Loarie et al. 2009), reveal the rate at which species have to move to maintain constant climate conditions. This measure is regularly used to examine the pressure that species face under rapid climate change (Burrows et al. 2014). In combination with other examinations of climate space it can reveal areas that may serve as climate refugia (Carroll et al. 2015; Roberts and Hamann 2016). Climate velocity is an important concept in corridor ecology, because it is assumed that minimizing velocity along a corridor increases the likelihood that species can use it for a longer period of time (Dobrowski and Parks 2016).
Climate refugia refers to places with lower climate velocity relative to the surrounding landscape. These areas can emerge on cooler aspects, areas adjacent to deep lakes or oceans, deep valleys that harbor cold air, streams fed by cold groundwater from deep aquifers, dense canopy cover, and topographically complex terrain with a diversity of microclimates (Morelli et al. 2016). Species are expected to persist and reproduce in climate refugia for a longer time than in the surrounding landscape. Populations may not always persist until the climate cools again and they may eventually need to move to more distant sites, but local refugia may afford them more time before longer range shifts will be required. Conserving refugia is now a recommended approach for planning climate-resilient protected area networks (Keeley et al. 2018; Keppel and Wardell-Johnson 2015). Shoo et al. (2013) further define internal refugia as climatically stable spaces within a species’ existing range as compared to external refugia found outside present ranges.
Riparian areas can sometimes serve as local refugia from warmer temperatures and drier conditions due to the cooler and moister microclimates that come with proximity to freshwater streams and wetlands. Riparian corridors are known to serve as movement corridors for many species (e. g.,Hilty 2001). Fortunately, in many places stream corridors have some legal protection in part because they provide protection for freshwater quality and quantity as a critical ecosystem service (Fremier et al. 2015).
Does structural connectivity equal functional connectivity? Structural connectivity may exist without functional connectivity. Here, we define the latter as the degree to which corridors actually facilitate or impede the movement of organisms. Some of the primary reasons for the disconnect between structuraal and functional connectivity are species’ dispersal behavior and abilities, the need for specific habitat requirements, and particular barriers. Some species may have traits that make functional connectivity between patches essential to their persistence. This can be the case for meta-populations that require recolonization from other emphemeral populations nearby especially when their habitat is degraded (Binzenhofer et al. 2008).
Dispersal. Dispersal, the process of individuals leaving the place where they are resident in search of a new place to live, is a key process to be considered in connectivity planning, because it greatly influences the demographic and evolutionary dynamics of populations (Stenseth and Lidicker 1992). There are several reasons why dispersal behaviors may prohibit species’ use of corridors and other landscape elements that provide structural connectivity. Species with poor dispersal abilities may not be able to move through long corridors that lack adequate water, food resources, and shelter. Behavioral factors such as avoidance of edges and disturbed habitats may limit species movements (St. Clair et al. 1998). Tropical forest understory birds especially are known to avoid crossing even narrow gaps in the vegetation (Machtans et al. 1996; Castellon and Sieving 2006). Dispersing animals may avoid territorial conspecifics and therefore be unable to use landscape elements that provide structural connectivity. Dispersing juvenile male lions (Panthera leo) avoided habitat preferred by adult lions, and instead used areas with high anthropogenic risks (Elliot et al. 2014). Mutualistic relationships and antagonistic relationships may also influence corridor use (Lidicker and Koenig 1996). For example, the spread of mistletoe depends on the behavior of mistletoe-eating bird species (Norton et al. 1995), and brown-headed cowbirds (Molothrus ater) parasitize nests of songbirds especially in edge habitats such as narrow corridors (Hansen et al. 2002). The process of dispersal in highly social species can be suppressed by the high risk of individuals moving solitarily and the difficulty of prospective immigrants to integrate into existing social groups. Thus, even in the presence of structural connectivity, habitat patches may remain unoccupied by animal species that are highly social (Cockburn 2003; Laurance 1995).
Habitat requirements. Habitat quality may determine whether structural connectivity equates to functional connectivity. For example, lemuroid ringtail possums (Hemibelideus lemuroides) were only found to use primary rainforest corridors of at least 200 m in width (Laurance and Laurance 1999). In highly modified environments, the habitat within a corridor may be disturbed, invaded by exotics, or sparsely vegetated. Non-native vegetation can render low habitat quality. For example, where Arundo donax and Tamarix chinensis have invaded riparian areas, some native species may avoid moving through the riparian corridor (Boose and Holt 1999; Stromberg 1997). Some arboreal species require corridors to have characteristics of intact forests and avoid them otherwise (Laurance 1995). Thus, what may be a corridor for some species does not fulfill this function for others. In particular, some species will need to reside within large habitat linkages to eventually move through them. If their specific habitat needs are not met they will not be able to persist within the corridor (Doerr et al. 2010). This will lead to generalist species, such as those that use multiple habitat types or have broad diets, to pass through, while for specialist species the landscape will still be fragmented (Dijak and Thompson 2000). Protecting wide swaths of land for connectivity and managing them for biodiversity can ensure that the specific habitat needs of specialist species are met. It will also minimize edge effects which can preclude species that primarily inhabit interior habitat from living in or moving through structural connectivity elements (e. g.,Mills 1996; Perault and Lomolino 2000).
Less obvious barriers to movement. Modeling and mapping the most likely pathways that species may use to move through a landscape based on structural connectivity is now relatively commonplace. For example, within the GuidosToolbox there is a module called “Reconnect” that can be used to detect stepping stones and quantify connected area gained by including corridors (Vogt and Riitters 2017). However, these models can fail to take into account what may seem like minor structures in the landscape that have enormous impacts on one or more focal species. For example, secondary roads may be a death trap for western toads (Anaxyrus bufo) where significant portions of populations may die crossing a busy road (McCrory and Mahr 2016). Other human structures and changes to the environment such as, but not limited to, fencing and canals, light, and noise can also inhibit movement of some focal species (e. g.,Tuxbury and Salmon 2005). There are also attractants to consider such as the placement of salt licks that can influence the course of species movements and preditor-prey interactions (Gonzalez et al. 2017; Lazarus et al. 2021).
Another consideration related to humans is whether their presence and management turns habitat from a source habitat where reproduction exceeds mortality to sink habitat where the reverse is true and populations may become extirpated in areas that a landscape model might predict as providing high-quality habitat connectivity. Just one house per section (square mile) on average transforms that land from source habitat where grizzly bear (Ursus arctos horribilis) could reproduce successfully to sink habitat where a bear is most likely to die (Schwartz et al. 2012). Rural low-density development creates source and sink dynamics for some birds as well (Hansen et al. 2005). Sink habitat can also be created where domestic animals or human-habituated wildlife flourish, causing dynamics like meso-carnivore release, the expansion of a smaller predator following the reduction or removal of a larger predator, that can have an impact on the survival of some native species (Brashares et al. 2009). To better predict and understand such scenarios, one requires a detailed understanding of animal behavior related to the built environment.
For some large carnivores, wildlife-human conflict is a threat to movement and species persistence (Primm and Clark 1996). In the Greater Yellowstone Ecosystem, most grizzly bears are killed by people (Haroldson and Frey 2002). To reduce this toll, co-existence efforts are employed that range from removal of livestock carcasses, the use of range riders (humans on horseback to reduce carnivore kills), bear-proofing dumpsters and trash, public education programs, and much more. The theory is that these tools will help humans and carnivores share landscapes, but more research is required to test the efficacy of these various strategies (Eklund et al. 2017). Understanding human resistance or fear of different species can help practitioners either choose to advance connectivity areas in places with lower human resistance or advance work seeking to change human values and resistance (Ghoddousi et al. 2021).
Methods and Results
Which traits render species vulnerable? Why are some species far more vulnerable than others? There is increasing reliance on trait-based analysis of species and communities in part due to the importance of predicting species loss, how well various interventions may lead to recovery, and the difficulty of obtaining detailed life history information for the myriad species at risk. For example, smaller range size is useful in predicting a species’ vulnerability to extinction along with narrow habitat breadth, small population size, and poor dispersal (Beissinger 2000). Species that avoid using or moving through modified habitats have less stable populations as was observed for non-flying mammals in Australia’s tripical rainforest (Laurance 1991). A recent meta-analysis of 173 manuscripts examined which well-studied traits may be predictors of extinction risk using any variables that indicated decline of the species over time (Chichorro et al. 2019). Mammals and birds were the most commonly studied groups; far fewer studies were done on fishes, insects, and plants. Body size and geographic-range size are frequently associated with rarity and, not surprisingly, these two variables, as well as habitat breadth, were most commonly included in predictive models. While positively correlated, the relationship between the two is not tight enough for one to be a surrogate for the other. While big animals are found in lower densities and generally have larger ranges, the range sizes of small-bodied animals vary from small to large (Gaston and Blackburn 1996). Findings from the meta-analysis reveal that geographic range size and specific habitat requirements are significant predictors of extinction risk. Body size, while most commonly studied, was not clearly linked to extinction risk. Generation length was also commonly included in these studies but found to be significant in only 27 % of the cases. Far less frequently examined was dispersal ability, which, however, was significant in 43 % of the relevant studies. The more common inclusion of body size and geographic range size reflects the fact that these data are easier to obtain than dispersal ability.
These same traits relate to species’ abilities to shift their ranges in response to climate change. Dispersal is particularly poorly described for most species and even less is known about long-distance dispersal that can be less frequent but very important in predicting colonization probability and range shifts. However, established demographic analyses of simulated species, parameterized with a wide range of values for a set of traits, are useful for exploring range shifts (Santini et al. 2016) and species persistence (Kitzes and Merenlender 2013). In simulations, median dispersal distance turned out to be the best predictor of species-spread rates with little power detected for annual survival rate, age of sexual maturity, litter size, or number of litters per year (Santini et al. 2016). The Santini et al. (2016) study also examined the utility of body mass, home range area, and population density as a proxy for other trait combinations to model the rate of spread and ability to shift species’ range location under climate change. While the rate of spread tended to increase for the first two variables (body mass and home range area) and decrease with the latter (population density), none of the traits proved to be a strong predictor of species’ spread rates (R2 = 0.3 for each).
There is strong theoretical support that greater dispersal ability will facilitate future range shifts, which will be necessary for many species to adapt to climate change. However, results from the simulations conducted by Santini et al. (2016) caution that the rate at which most of the virtual species they simulated are estimated to move is slower than the global mean velocity of climate as predicted by Loarie et al. (2009). A meta-analysis of empirical research on 26 taxonomic assemblages conducted by MacLean and Beissinger (2017) revealed that historic range limit was the strongest predictor variable (expressed for 60 % of the studies) for limited range shifts. Assemblages found at higher elevations and latitudes demonstrated smaller range shifts than those occupying lower locations. Range shifts increased with habitat breadth when differences in study area size were accounted for and a positive relationship for movement ability (related to dispersal) was observed for 50 % of the studies. No overarching significant effect among these studies was detected that indicated an influence of body size, fecundity, or diet breadth on range shifts.
Reflecting on this work, the most vulnerable species given the pressures of climate change are likely to have limited dispersal capacity, restricted ranges, and strong habitat specificity (or narrow habitat breadth) - traits that make them susceptible to habitat loss and fragmentation especially. To persist, these species likely need special consideration to ensure functional habitat connectivity.
Species viability and persistence. Species persistence is the raison d’être of corridor conservation. Incorporating species persistence into protected-area-network design relates back to metapopulation theory, where groups of subpopulations need to remain linked by dispersal between patches and maintain some gene flow. Measures of persistence include mean time to extinction or probability of extinction within a given timeframe. These measures are generally estimated by modeling species viability based on species-specific life-history information (e. g., birth, death, immigration, and emigration rates). Estimates of species viability rely on measures of life-history, demographic, and ecological information to quantify extinction risk (Beissinger and McCullough 2002; Shaffer 1981). This same approach is also used to estimate the influence of environmental stochasticity and resource extraction or land management on species persistence (Akcakaya et al. 2004; Wintle et al. 2005).
Improving persistence may result from maintaining or creating corridors that allow for continued dispersal between habitat patches to lower species-extinction probabilities and reduce genetic degradation, yet species persistence measures are very rarely assessed as part of corridor design or planning. Incorporating species-viability analysis into corridor planning requires a spatially explicit approach that combines traditional population-viability analyses with a GIS that includes attributes that influence the model parameters, such as the impact of habitat type on reproductive and dispersal rates. This approach was taken for a tiger (Pantheris tigris sumatrae) subpopulation in a region in Sumatra, Indonesia: A population viability analysis was performed to assess the importance of corridors for the persistence of the different populations under different levels of poaching pressure (Linkie et al. 2006). Their models indicate that maintaining connectivity between the largest core area and a smaller core area would greatly improve the long-term persistence of tigers in the region.
Maximizing persistence using a persistence-like index based on the probability of occurrence and colonization was used for European mink (Mustela lutreola) and a water clover (Marsilea quadrifolia; found on the Iberian Peninsula) as one approach to evaluating spatial linkage design outcomes (Alagador et al. 2016). One key result from this study is that when implementation cost is not overly constraining algorithms that maximize persistence provide the best-performing model for designing ecological networks for conservation.
The approaches we are advocating here follow Bill Lidicker’s perspective on the importance of species ecology and the need to know the role that species corridors are intended to serve before we can design and implement corridors, or assess their effectiveness. However, it does not address the problem we raise about the lack of available ecological information to properly parameterize focal-species movement or connectivity models. Incorporating species viability into corridor planning and assessment requires a good deal of data for a single species, making these methods infeasible for applications to large numbers of species. An important way to address this constraint is to develop spatially explicit, stochastic, demographic metapopulation models that can be parameterized for many species (Nicholson et al. 2006).
Discussion
New research directions. Given the lack of readily available species-ecological data and the high cost of obtaining this information, we recommend using species traits, considerations around population persistence, and vulnerability to climate change to identify which species should be studied to determine if a proposed connectivity-conservation plan is likely to function as intended for more vulnerable species.
With new technologies (e. g.,Kays et al. 2015), collecting extensive movement data for many species is becoming possible. Movement-path data will increasingly become available for planning and justifying costly on-the-ground implementation action. Learning more about how organisms move through landscapes during daily movements, migrations, and dispersal, the cues they use to navigate, and the resources that are critical for their safe passage will improve our ability to design effective corridors. When direct observations of animal movement paths are not available for a planning site, applying statistical models to animal-movement data from nearby can be a good way to link connectivity evaluations to empirical data. Movement data are also ideal for generating public enthusiasm for a corridor project (Keeley et al. 2018; Morrison and Boyce 2009). Social-science studies on how knowledge of individual animals’ movements influences people’s willingness to protect and respect wildlife corridors are needed.
A critical question that has not been answered yet is how wide corridors should be to meet conservation objectives. Beier (2019) gives the rule of thumb that a width of 2 km is appropriate when the corridor is intended to connect protected areas up to 80 km apart in a landscape likely to experience impact from development in the future. However, empirical evidence is needed to help planners and managers decide on the appropriate corridor width in different scenarios.
With climate change, species that are not commonly considered in connectivity plans will need to shift their ranges. Research and monitoring are necessary to determine which species’ connectivity needs are not met by structural connectivity and will need special attention (e. g., (Carroll et al. 2018). It will also be critical to understand when the velocity of climate change exceeds the dispersal ability of species potentially requiring assisted migration.
Once corridors are established and/or protected, monitoring becomes essential to allow for adaptive management, which is a science-based, structured approach to improving our understanding and reducing uncertainties. Systematic monitoring and adaptive management need to occur to increase the likelihood that ecological corridor projects will meet their objectives, and that we learn from corridors that have been implemented. Monitoring is especially necessary to determine for which species coarse filter corridors do not meet connectivity requirements. In addition to documenting movement paths, camera-traps are an excellent tool for detecting medium- to large-sized animals especially in narrowly vegetated corridors (LaPoint et al. 2013) and crossing structures (Ng et al. 2004). Roadkill distributions, winter snow or sand-tracking transects, track plates, scat surveys with or without scat-detecting dogs, and bird surveys are other ways wildlife biologists or local naturalists can determine whether focal species are using a corridor (Merenlender et al. 1998). Long-term monitoring is essential to understand how species with restricted ranges respond to climate change. Are connected landscapes adequate to allow these species to shift their ranges with climate change? Answering this question will require presence data collected at fine resolution across broad spatial scales over many years. Crowd sourcing can be an efficient approach to obtain these types of data (Fink et al. 2014).
Even when habitats are connected structurally, their populations can remain susceptible to local extinction because gene flow and recolonizations cease between habitat patches that are not functionally connected. We discuss several reasons for this, including the complexities of dispersal, specific habitat requirements not reflected in land-cover data, and less obvious barriers to movement. Both theoretical and empirical evidence suggests that movement or dispersal ability, geographic-range restrictions, and habitat restrictions predict the potential for species to shift their ranges. Assessing focal species for these particular traits is one way to determine when to examine functional connectivity using established demographic analyses and modeling.
Rapid climate change is forcing a new approach to ecological connectivity to accommodate longer-term species-range shifts including protecting climatic refugia, connecting climatically analogous areas, and protecting corridors that follow similar climate types. The focus on physical environmental data for climate-wise connectivity including climate velocity, analogs, and refugia is in line with structural connectivity approaches. However, there is little evidence that these structural approaches will lead to functional connectivity for the reasons we outline above. Given the requirement for more detailed species data especially on dispersal, which is difficult to obtain, we suggest prioritizing species-based data collection and modeling on the species most vulnerable to habitat loss and climate change.
Finally, new technologies to monitor individual movement and species utilization of corridors varying in width and other characteristics will help managers plan and verify functional connectivity. Engaging the public in contributing data is a way to improve demographic data collection required to assess persistence and the impacts of protecting patches and linkages across large-scale ecological networks. In sum, research into species with restricted ranges and low dispersal capacity is crucial if we are to design resilient ecological networks that will also serve the most vulnerable species.