Introduction
Among its extensive field of application that includes the development of batteries, fuel cells, supercapacitors and environmental remediation devices, electrochemistry has become a well-established topic of interest in academic and educational fields after the growing and constant development of electrochemical sensors (Eliaz y Gileadi 2019). Current electrochemical sensing systems present different structures whose properties allow professionals to quantify chemical, biological, and environmental parameters (Zhang et al. 2020; Kim et al. 2019; Kang et al. 2017). These devices, due to their prevalent application in healthcare are mainly focused on analytes such as oxygen (Rivas et al. 2020; Vaneev et al. 2020), glucose (Peng et al. 2021, Espro et al. 2020) and hydrogen peroxide (Patella et al. 2021; Dang et al. 2020; Garate et al. 2018) which are involved in several biological processes. Among the analytes, hydrogen peroxide sensing is one of the most reported in the literature as its determination does not require controlled measurement conditions like an inert atmosphere, implying that preliminary operational and performance laboratory tests can be performed with the simple preparation of a stock solution.
Due to the applications, an increasing number of practical/theoretical proposals regarding basic electrochemistry concepts and introductory electrocatalytic devices have been published as novel didactic resources for undergraduate/graduate levels (Elgrishi et al. 2018; Hendel and Young 2016; Saxena and Stasangee 2014). Previously referenced works set a solid foundation to continue expanding electrochemistry’s relevance throughout both graduate and undergraduate curriculum. Thus, an experiential practice that allows students to perform quantitative H2O2 electrochemical sensors characterization and performance validation through well-known analytical figures of merit (Justino et al. 2010) is a proposal that presents both continuity and conceptual enrichment, although certain implementation considerations must be considered.
Firstly, electrochemical sensing strategies to detect H2O2 dissolved in an electrolyte buffer differ according to the working electrode’s (WE) conformation. For example, first generation sensors (Gulaboski et al. 2019, Lyu et al. 2019; Sarhan et al. 2019; Yang et al. 2018) based on bare metal WE with nanoparticles attached to it differ completely from third generation ones (Xu et al. 2021; Soto et al. 2021; Gulaboski et al. 2019, Waifalkar et al. 2018), which present a sandwich structure containing a conductive surface, attached nanoparticles with an immobilized redox mediator and a H2O2 selective metalloprotein. This structural complexity translates into its functionality analysis as the presence or absence of interfacial nanomaterials and additional redox mediators can transform a simple solution electrode transfer process into a redox cascade mechanism. Secondly, the previously detailed differences between sensor generations have an impact on the instrumental demands, where the accessibility to nanomaterials, enzymes or surface functionalization reagents in laboratory courses may be limited. As a result, designing laboratory experiences involving electrochemical sensing should consider its conceptual complexity while minimizing the instrumental demands as much as possible, if one desires to guarantee its accessibility to broader students’ demographics.
This work presents an experiential learning proposal that allows students to understand from a theoretical and practical perspective the core concepts of quantitative electrochemical sensing characterization and performance analysis through obtaining analytical figures of merit, using an H2O2 sensitive low-cost ferrite powder first generation electrode. The proposed laboratory protocol is suitable for graduate/upper-level undergraduate physical or analytical chemistry courses and was designed to be conducted in groups of two to three students over a four-hour period, allowing the completion of theoretical background exposition, hands-on experience, and a closing questionnaire.
Equipment and Materials
All chemicals were of analytical grade and used without further purification. Using a three-electrode electrochemical cell scheme, a Pt foil with an area of 5 cm2 was used as the counter electrode (CE) and a silver/ silver chloride electrode (Ag|AgCl|1 M KCl) was used as a reference electrode (RE). Polyvinylpyrrolidone (PVP K30, Anedra, Argentina) and a commercial, technical grade ferrite powder (Pilar Aquarel, Argentina) mainly composed of a mixed iron oxide (Fe3O4) were used for the preparation of the working electrode (WE). Ferrite powder dispersions were mixed with a 20% w/v waterborne PVP stock solution to obtain a ferrite to PVP mass ratio of 1:10 and the ferrite/PVP formulation was drop-casted onto either a glassy carbon (GC) or platinum (optional) rotating disc electrode (5mm diameter) which were dried overnight at room temperature or after 5-10 minutes using a portable electric space heater.
Electrochemical voltammetric and amperometric measurements and data acquisition were carried out using a Teq4 potentiostat (NanoTeq, Argentina), while data processing was performed using OriginLab.
Hazards
All chemicals used are harmful if swallowed and cause eye and skin irritation on contact. H2O2 is an oxidizer, unstable and light-sensitive reagent so it must be stored in a refrigeration unit, away from the other chemicals. If a refrigeration unit were unavailable, any dark, cool, and dry space will suffice during the development of the experimental protocol. Under these considerations, students must read the respective reagents safety sheets via their preferred material safety data sheet (MSDS) database to guarantee their proper handling and are required to bring personal protective equipment including a lab coat, safety goggles and disposable latex gloves, which must be worn during the whole experimental procedure.
Reported Results Considerations
As stated before, reported experiments were carried out drop-casting 10 μL of a ferrite/PVP formulation onto a GC rotating disc electrode (5 mm diameter). Both working electrode’s face up and face down schemes are suitable to reproduce the proposed protocol without any issues. In case of wire-like working electrodes, further experimentation should be conducted to formulate a ferrite/PVP composite whose viscosity level guarantees both adherence to the electrodes’ surface and stability during the measurement process. Furthermore, considering the possible variability in the composition of commercial ferrites, the reported values may differ, requiring modifications in parameters such as the concentrations of hydrogen peroxide and interferents. Due to the aformentioned considerations, it is recommended to carry out a test run of the experiment before conducting the laboratory work with students.
Laboratory Protocol Overview
Figure 1 illustrates the overall scheme of the laboratory protocol which can be separated into two sections. The theoretical section (Fig. 1A) consists of a brief review of several electrochemical techniques including cyclic voltammetry and chronoamperometry to introduce H2O2 electrochemical sensing. Lastly, both concepts converge when presenting from theoretical and operational perspectives the electrochemical performance validation techniques known as electroanalytical figures of merit.
On the other hand, the experiential (Fig. 1B) section proposes a hands-on experience where students are required to implement the acquired theoretical background and develop technical and analytical skills regarding electrochemical sensors’ figures of merit. This section contains an additional resource called “Windows of Opportunity” (WO), which contains additional activities to conceptually enrich the laboratory experience and are put to the teacher’s consideration. Further guidelines about this additional resource can be found in the Supporting Info - Laboratory Protocol with Questionnaire Solutions file.
Theoretical Section
Designing this didactic proposal as a continuation of Hendel and Elgrishi works (Elgrishi et al. 2018; Hendel and Young 2016), students are required to present a solid background knowledge on electrochemical cells, cyclic voltammetry, electrode potential scanning and coupled chemical reactions. In case of a needed conceptual reinforcement adding extracts of Bard’s Electrochemical Methods: Fundamentals and Applications chapters 4 to 6 into the main texts is recommended (Bard and Faulkner 2001).
The introductory review focuses on two electroanalytical techniques, cyclic voltammetry (CV) and chronoamperometry (Behzad and Neda 2019; Choudhary et al. 2017), which measure faradaic current because of either oxidation or reduction processes of an electroactive analyte at the WE surface, allowing a quantitative and qualitative study to be carried out.
CV is one of the most common electrochemical techniques which measures current changes by sweeping fixed potential windows back and forth. The graphical information provided by CV (i.e., current vs. electrode potential plots called voltammograms) can be used to understand the WE basic electrochemical properties such as capacitive behavior and reversibility, or to identify anodic/cathodic redox peaks which imply that the analyte or material on the WE surface is going through oxidation and reduction processes respectively. On the other hand, chronoamperometry is a frequently reported amperometric method generally used alongside CV. In this technique, a pulse potential is applied to a working electrode establishing a fixed potential difference at the electrode/electrolyte interface and the associated current values are recorded versus time. Measured current shows fluctuations over time in response to the diffusion process of the analyte of interest at WE surface, allowing for an in-depth analysis of diffusion-controlled processes.
Before following with a general introduction of H2O2 electrochemical sensors, a moderate understanding of its redox chemistry and behavior is required. Under a neutral medium (pH 7) and versus a saturated calomel reference electrode (SCE), H2O2 two-electron half reactions can be described as follows:
The standard redox potential for the reduction of H2O2 to water [1] is considerably high. This, from a thermodynamic perspective, would imply that H2O2 can be reduced by a wide spectrum of materials. However, redox systems with H2O2 under neutral conditions are not thermodynamically driven but mainly kinetically (Winterbourn 2013). As a result, slow kinetics involved in electrochemical electron transfer leads to very high over-potentials during H2O2 sensing, and this fact is particularly evident at bare metal electrodes whose voltammetric profiles only show a single irreversible cathodic peak (Chen et al. 2013). Due to the kinetic restrictions, metal oxides and carbon structures are attached to the WE to accelerate the H2O2 redox process up to reversible or quasi-reversible conditions (Veiga et al. 2020; Zhang et al. 2011).
The development of working electrodes whose composition eases H2O2 sensing led to the design of three standard structures classified by Gulaboski as first, second and third generation H2O2 electrochemical sensors (Gulaboski et al. 2019).
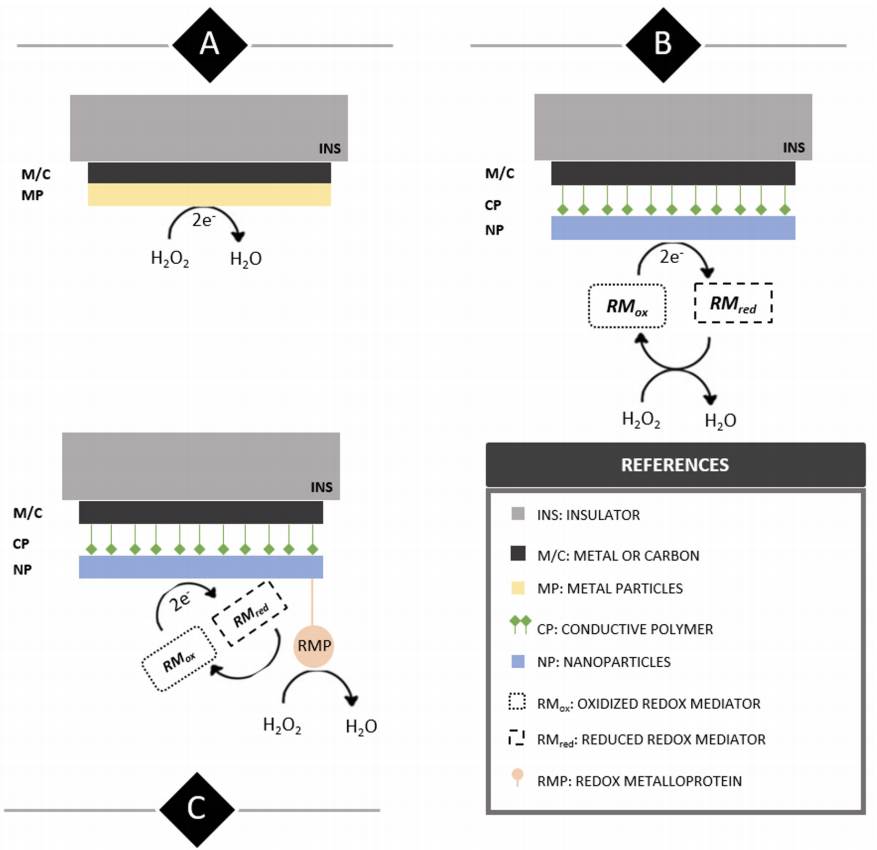
Figure 2 Illustrative side view display of structural and functional differences between first (A), second (B) and third generation (C) H2O2 electrochemical sensors.
First generation electrochemical H2O2 sensors’ working electrodes present successive layers of an insulator (INS) such as polytetrafluoroethylene (PTFE) and a bare metal or carbon surface (M/C) modified with the addition of metallic or metal oxide particles (MP) to increase the electroactive area and analyte sensitivity. From a methodological perspective, analyte quantification is pretty much straightforward and involves performing amperometric or voltammetric techniques in an electrolyte buffer containing H2O2 . On the other hand, second generation working electrodes present surface modifications including a layer of conductive polymer (CP) such as polyaniline (PANI) to which nanomaterials (NP) like multi-walled carbon nanotubes (MWCNT) easily attach and increase the WE electroactive area. In this case, measurements are performed indirectly by the presence of a redox mediator added to the electrolyte buffer (RM) which partakes in a redox cycle under the presence of H2O2 and transfers the process-resulting electrodes to the WE surface.
Lastly, third generation sensors share the same components as those of second-generation ones, but their surface is further functionalized by adding a redox metalloprotein (RMP) such as horseradish peroxidase with high selectivity towards H2O2. Analyte measurements are generally carried out at a fixed potential and quantification is indirect through an electron transfer cascade that starts with the enzymatic reduction of H2O2 and continues via electron transfer thus reducing the redox mediator close to the WE surface.
Once the electrochemical sensors’ structural and functional characteristics are presented the next criteria to develop is what parameters are indicators of its quality and performance. According to Luong for an electrochemical sensor to be effectively transferred into its respective fields of application the device must meet three criteria: adjusting costs, reducing technological barriers, and presenting an adequate analytical validation (Luong et al. 2008). In analytical chemistry, validation is an essential procedure to guarantee closeness between an analyte’s unknown true value and the results obtained from the proposed method. Electrochemical methods can be validated by assessing their figures of merit, which are quantifiable related both to the methodology and analyte of interest (Olivieri et al. 2006). For the present didactic proposal, the determination of the following figures of merit has been selected: sensitivity, reproducibility, repeatability, dynamic linear range (DLR), limit of detection (LOD), limit of quantification (LOQ) and selectivity.
Sensitivity and selectivity are parameters used for assessing an electrochemical sensor’s reliability. The sensitivity towards a given analyte is defined as the slope of the analytical calibration curve, while from a method perspective this concept can be interpreted as a signal change after an analyte concentration variation within a linear regime. This linear regime is known as the dynamic linear range and extends from the LOQ up to the limit of linearity (LOL) where the measured signal does not present a linear dependency with the analyte concentration anymore. On the other hand, selectivity is defined as the ratio of the measured signal between the analyte of interest and a particular interferent. Under these criteria, a method is selective when it can accurately quantify the analyte of interest under the presence of interferences, whose contributions to the measured signal are negligible.
Repeatability and reproducibility, in terms of electrochemical sensors, refer to the proximity between the sensitivity results obtained from successive measurements of the same analyte under the same (repeatability) or different (reproducibility) conditions related to operators and instrumental (McNaught and Wilkinson 1997). Finally, and as closure for the theoretical section, the LOD refers to the minimum detectable concentration for a given analytical procedure, while the LOQ is the lowest analyte concentration that can be quantitatively detected with a considerable degree of accuracy and precision.
Experiential Section
Methodologically speaking, the hands-on experience is divided into two sections. Firstly, students are required to perform a voltammetric study of the working electrode allowing both a basic understanding of the redox processes occurring at the WE surface and selecting the appropriate fixed potential condition to continue with the electrochemical sensing of the H2O2 reduction. Secondly, previously detailed figures of merit will be obtained and analyzed by students via chronoamperometry. The information obtained from these determinations will allow a conclusion to be drawn regarding the ferrite powder/PVP sensing performance towards H2O2 .
The voltammetric study begins as illustrated by Figure 3A where students compare the voltammetric profiles between a bare and a ferrite/PVP drop-casted glassy carbon electrode in 0.1 M phosphate buffer solution of pH 7.4 at a scan rate of 0.05 V s-1. A brief analysis of the graphical results shows that the ferrite/PVP electrode displayed an increased capacitive behavior due to its rectangle-shaped voltammogram and increased current values when compared to the bare GC electrode. Furthermore, the proposed WE shows an anodic peak around -0.1 V and a cathodic peak at -0.16 V, which considering the ferrite composition could be assigned to the oxidation of Fe2+ to Fe3+ and the further reduction of Fe3+ to Fe2+. These well-defined redox peaks allow students to construct a potential window map displayed in Figure 3B to select the appropriate chronoamperometry measurement conditions towards H2O2 reduction. In this case, an appropriate fixed potential selection is -0.25 V, as it is a constant region of the voltammogram where ferrite acts as a reducing agent, meaning that its preponderant redox species is Fe2+.
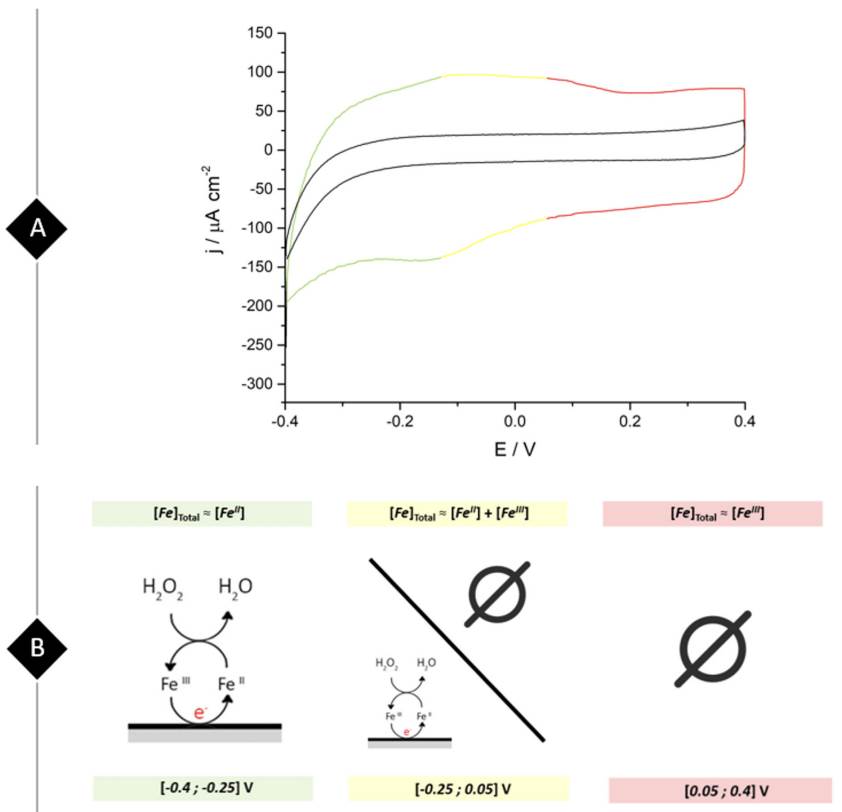
Figure 3 (A) Cyclic voltammogram obtained at a bare (black line) and ferrite/PVP (three-colored) drop-casted glassy carbon electrode in a 0.1 M phosphate buffer solution of pH 7.4 at a scan rate of 0.05 V s-1. (B) Potential-dependent working electrode redox behavior towards H2O2 reduction in correlation to the voltammogram colored regions.
WO #1: Voltammetric analysis of the ferrite/PVP working electrode can be further developed. The selection of the fixed potencial for chronoamperometry can be performed by recording several voltammetric profiles after the successive addition of 0.1 mM H2O2 to analyze the evolution of the cathodic peak.
Continuing with the amperometric studies, students begin by analyzing the WE sensitivity, repeatability, and reproducibility. Figure 4A shows ferrite/PVP WE current density-time curves in a 0.1 M phosphate buffer solution of pH 7.4 at -0.25 V after the successive addition of 0.2 mM H2O2 up to 1mM. This analyte concentrations window was previously tested to guarantee the desired linear current concentration response up to the LOL (check Supporting Info - Additional Figures for further graphic information), facilitating students’ curve-fitting and sensitivity values calculation, expressed as µA cm2 mM-1.
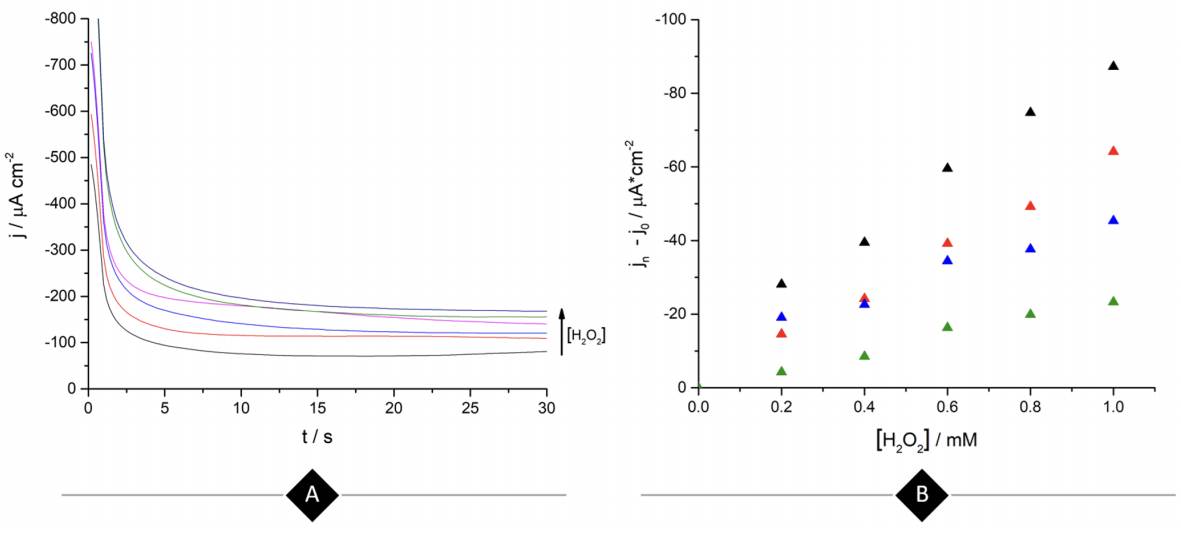
Figure 4 (A) Ferrite/PVP WE current density-time curves of 0.1 M phosphate buffer solution of pH 7.4 at -0.25 V after the successive addition of 0.2 mM H2O2 up to 1mM. (B) Repeatability of the ferrite/PVP WE studied by measuring the linear dependence of current density with H2O2 concentration at -0.25 V (Jn) adjusted by the blank’s current density values (Jo). Repetitions 1 to 4 are represented by black, red, blue, and green colored triangles, respectively.
Later, students perform repeatability and reproducibility validations by simply repeating (n=4) the determination either by using the same drop-casted WE (repeatability) or cleaning the glassy carbon electrode after each measurement and drop-casting a new ferrite electroactive layer (reproducibility). Figure 4A displays repeatability essay results, showing a linear dependence between current density measured at -0.25 V with H2O2 concentration which presents students with a case of a gradual sensitivity loss percentage up to 73% when comparing successive sensitivity values obtained from linear regressions (Supporting Info - Additional Figures - Table 2). This can be attributed to the dissolution of particles from the electrode to the buffer solution after consecutive measurements.
WO #2: Chronoamperometric results show a typical transient response profile to an applied potential step, whit the particularity that rather than the current falling off to zero as predicted by the Cottrell equation, measured current flactuates around a steady current value (Bard and Faulker 2001). This could indicate eigther slow WE kinetics or well-mixed bulk solution that preserves a constant concentration of electroactive species. Obtained chronoamperometric data can be further analyzed from a theoretical transport/kinetic perspective.
To obtain the LOD and LOQ, students follow a simple signal-to-noise ratio procedure measuring the 10 chronoamperometric blank determinations at -0.25 V, which in this case refers to 0.1 M phosphate buffer solution of pH 7.4 and then perform a simple mathematical operation [3-4]. Here SD refers to the standard deviation of the blank determinations and m to the slope of the analyte calibration curve. With the figures of merit obtained so far and considering 1mM of H2O2 as the limit of linearity, students will be able to report the dynamic linear range (Miller and Miller 2005).
Consecutively, students carry out selectivity studies by comparing the current-potential curves of commonly reported interferents (He et al. 2019; Meng et al. 2015) such as KCl + Na2SO4, ascorbic acid and glucose against the analyte of interest (Fig. 5). For this essay to be representative the tested interferents’ concentration was ten times higher than that of H2O2 and sensitivity results were expressed as a ratio between the current density changes after the addition of 2mM of the tested interferent and 0.2 mM H2O2 [5].
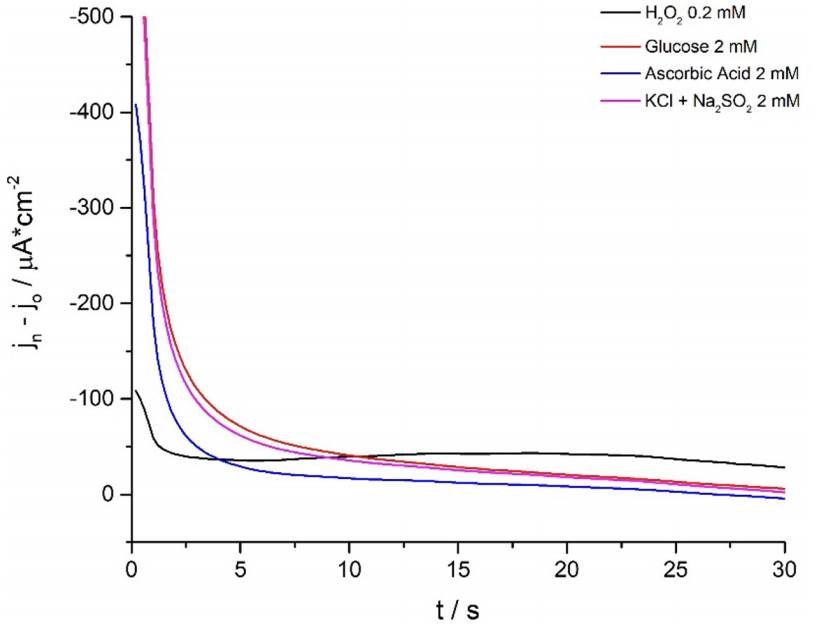
Figure 5 Ferrite/PVP WE current density-time curves of 0.1 M phosphate buffer solution of pH 7.4 at -0.25 V after the addition of 0,20 mM H2O2 (black line); 2,0 mM of KCl + Na2SO4, ascorbic acid and glucose (magenta, blue and red-colored lines, respectively). Results are expressed as the current density difference before (Jo) and after the addition of the respective analytes (Jn).
Finally, the different groups of students share a brief report containing obtained parameters (Table 1), making comparisons, and analyzing possible differences with their peers. This simulated interlaboratory situation constitutes a space where students can discuss theoretical and technical aspects regarding the performed measurements, while a brief conclusion about the tested working electrode can be elaborated. As shown in Table 1, the proposed ferrite/PVP electrochemical sensor presents highly reproducible sensitivity values but due to the interference analysis and repeatability results its use is limited to one-use for samples previously treated for common interferences. Additional graphical information associated with the reported values is available in the Supporting Info - Additional Figures.
Table 1 Data report corresponding to the analytical figures of merit of a drop-casted ferrite/PVP glassy carbon working electrode.
FERRITE/PVP ELECTROCHEMICAL SENSOR - ANALYTICAL FIGURES OF MERIT REPORT | |||||||
---|---|---|---|---|---|---|---|
Sensitivity / µA cm2 mM-1 | LOD / mM | LOQ / mM | DLR / mM | Selectivity | |||
Reproducibility | Repeatability | KCl + Na2SO4 | Asc. Acid | Glucose | |||
90.80 ± 10.63 | 54.81 ± 28.21 | 0.04 ± 0.01 | 0.13 ± 0.03 | 0.13 - 1 | 0.08 | 0.15 | 0.21 |
WO #3: Taking advantage of the acceptable H2O2 sensing performance of the electrochemical sensor studied, the proposed laboratory practice can be further contextualized through real-world applications. With this purpose, an additional activity consists of testing the ferrite/PVP electrochemical H2O2 sensing capabilities in commercial samples such as pharmaceutical hydrogen peroxide as a validation procedure towards reported concentrations.
Conclusion
Through the selection of electrochemical sensing as the topic of interest, this work responds to the need of contextualizing laboratory practices through real-world applications to hopefully provide teachers with instrumental, theoretical, and didactical resources to accompany their endeavor to implement electrochemical practices in both graduate and undergraduate curriculum. Presenting an integrative approach, comprising a theoretical introduction, experiential practice and post-lab results discussion, this protocol expects to facilitate the understanding of quantitative electrochemical sensing characterization and performance analysis. Basic electroanalytic concepts that students will be able to transfer effectively while performing future electrochemical practices.