Introduction
Why should one find a particular set of small mammals at any particular site? This question is at the heart of community ecology and has been investigated by many ecologists following on the ideas set out by MacArthur and Wilson (1963, 1967). While no definitive and conclusive answer can yet be provided, this paper does summarize a body of research that has explored, and I think clarified as my research matured, some of the factors that influence which species occur where and when. The important role that assembly rules might play in restricting which sets of species actually co-occur was first examined by Jared Diamond (1975), using the distributions of bird species in New Guinea and adjacent islands to study how they were assembled into communities. Diamond observed combinations of species of birds, existing in nature, which he termed ‘permitted’ combinations, and others that have not been found to exist in nature, which he termed ‘forbidden’ combinations. He said as part of his summary “Much of the explanation for assembly rules has to do with competition for resources and with harvesting of resources by permitted combinations so as to minimize the unutilized resources available to support potential invaders” (Diamond 1975:345). Although there has been much controversy, these views were supported a decade later by many chapters in an edited book on community ecology (Diamond and Case 1986). Bob M’Closkey elegantly demonstrated one mechanism that could explain how Diamond’s assembly rule might operate, making use of information on the diets of seed-eating rodents in the Sonoran Desert to describe in detail both their habitat niche and food niche (see M’Closkey 1978, 1985). Morris and Knight (1996) later provided a theoretical derivation for assembly rules as a probabilistic consequence of MacArthur-(Tilman 1982).
Many factors that have been related directly to mammal species richness at local scales (e. g.,Fox and Fox 2000) could also be related to landscape ecology (see Lidicker 1995). Lidicker (2008) proposed the term “ecospace” for a fourth level of ecology, to replace “landscape ecology”, rather than other possible terms such as “spatial ecology” or “ecosystem ecology.” Lidicker (2008) suggested “ecospace” after writing a review (see Lidicker 2007) for a book that assembled 37 foundational papers ranging from 1915 to 1990 that led to the emergence of landscape ecology as a discipline. Corridor ecology examines animal movements and is a subdiscipline of landscape ecology, which examines species distributions. Bill Lidicker co-edited two books of contributions to the subdiscipline of corridor ecology (see Hilty et al. 2006, 2019).
This paper explores in detail several factors, such as habitat selection, ecological succession, species interactions, and species assembly rules, which can have an impact upon small mammal communities. My focus here is on insights into these factors that can be gained by examining a series of studies initiated from a main study site at Big Gibber, a specific location within Myall Lakes National Park (MLNP), almost 300 Km north of Sydney, New South Wales, Australia (see Figure 1). ‘Gibber’ in this sense is an Australian term meaning a stone, so this location really means ‘big stone’. The natural disturbance of wildfire upon heathland and open eucalypt forest sites is compared to the disturbance caused by sand mining on these same landscapes, and the regeneration that follows these disturbances. Comparisons with other studies in similar landscapes illuminate how these factors influence the landscape ecology of small mammals.
Materials and methods
Study site. Sand dunes are important components of Myall Lakes National Park (MLNP; 32° 28’ S and 152° 24’ E; see Figure 1) located 300 Km, by road, north of Sydney in New South Wales, Australia. Outer barrier sand dunes up to 100 m high support 25-m high eucalypt open forest, whereas low-relief inner barrier sand dunes support wet and dry heathland. The four to five meters of relief provided by these low inner barrier dunes permit gradation from swamp to tall dry heath (Osborne and Robertson 1939; Carolin 1970). Such a gradation occurs on this Big Gibber main study site, part of a former lagoon that now supports swamp and wet heath in some areas, with a low transverse dune supporting dry heath and a patch of eucalypt woodland with a dense, tall, dry heath understory, thus including the full range of habitats available. Names for types of vegetation (macrohabitat) used in this paper and other papers cited follow the protocol established by Specht (1970, 1981). The main study site (approximately seven ha, adjacent to a gravel road constructed by the sand mining company) near Big Gibber (see Figure 2) is located in coastal heathland that had not been burned for a long time.
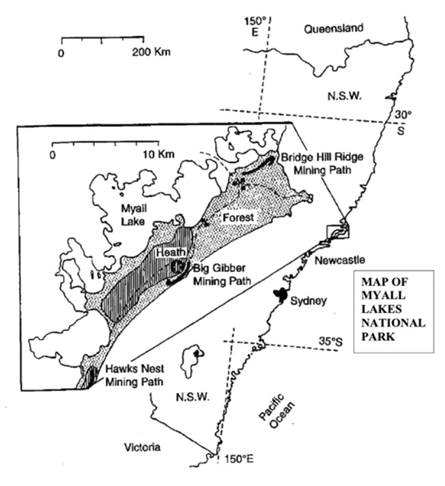
Figure 1 A location map of Myall Lakes National Park, almost 300 Km by road north of Sydney, in coastal New South Wales (NSW) with latitude and longitude lines shown. The main study site at Big Gibber is shown as a star. Mining paths are shown as heavy dark lines at Hawks Nest, Big Gibber and Bridge Hill Ridge. Areas of heath (shading) and forest (stippled) are shown together with larger solid dots in the forest representing trapping plots used by Fox and McKay (1979). This figure has been adapted from a figure published by Fox (1996).
This paper is a synthesis of many studies by the author and colleagues and full details of methods are included in the references cited, with a representative summary of those methods included here. A trapping grid with 20-m trap spacing was established in May 1974 and topography was mapped using a theodolite and staff to measure and record elevation above an arbitrary datum (see Figure 2). The grid was trapped at monthly intervals using collapsible aluminum Elliott small mammal traps (330 x 100 x 90 mm; Elliott Scientific Equipment, Upwey, Victoria, Australia) placed singly on the ground at each of 176 trapping stations, baited with a mixture of oatmeal and peanut butter. For each trapping session in May, June, and July of 1974 trapping was carried out on four successive nights with no pre-baiting. All but approximately one ha of this main Big Gibber study site was burned during an extensive wildfire in August 1974, which burned in total more than 2,000 ha of MLNP. Post-fire trapping sessions were carried out at intervals of two months on this site, as for several months there were no small mammal captures at all. More complete details of methods used on this site have been published (see Fox 1981, 1982).
To study vegetation regeneration and recolonization by small mammals following sand mining that occurred nearby, a number of 1-ha study plots were established, each with 25 traps set on a square grid with 20-m spacing between trap stations. Plots were established along a mining path at positions of known age since regeneration began. Such use of chronosequence analysis has since been validated for sand mining paths through eucalypt open forest (Twigg et al. 1989). To study heathland that had been sand mined, eleven study plots of known regeneration age were established along a sand mining path through heathland near Hawks Nest at the southern end of MLNP (see Figure 1). Three additional control plots were also established at adjacent unmined sites (see Fox and Fox 1978). Collection of vegetation survey information and small mammal trapping information was standardized across all of these study plots to be the same as for the studies that had already been undertaken on the main Big Gibber site. For a study of forest that had also been sand mined, we established a total of 20 study plots of known regeneration age (see Fox and Fox 1984). Eight were on one mining path (Big Gibber mining path) and twelve were on another (Bridge Hill Ridge mining path; see Figure 1). For more complete details of all methods used in these two studies, see Fox and Fox (1978, 1984). Control plot information for sites not mined came from 16 forest sites, near Seal Rocks (see Figure 1), within MLNP (see Fox and McKay 1981). This information allowed direct comparisons of results for the regeneration of vegetation and the recolonization by small mammals following wildfire and following sand mining.
Cluster analysis and factor analysis were used with vegetation density measurements (microhabitat) from different vertical layers, from ground level to 2 m above ground level, to identify vegetation types present on each site. Multiple regression analyses using a standard step-wise process were used to identify which variables contributed most notably to the abundance and biomass of small mammals present. In every case, appropriate statistical tests were used to measure significance to a level of at least P < 0.05. These tests were identified and explained in the publications cited for the data used from these various studies. Complete and detailed methods for all of these techniques, used on species in both wet and dry heath habitats as well as open forest habitats have been published (Haering and Fox 1995, 1997; Knight and Fox 2000; Ross et al. 2004).
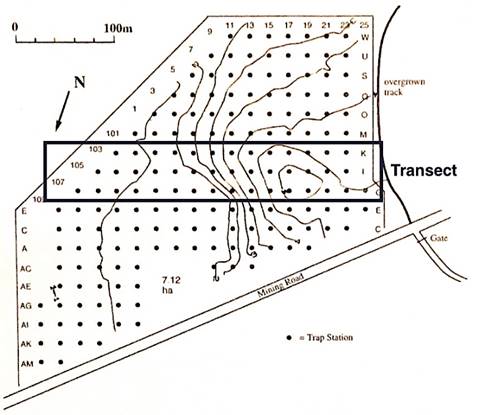
Figure 2 Topography on the Big Gibber study site in Myall Lakes National Park (MLNP) showing contours with a 0.5 m interval. The site is adjacent to a gravel road constructed by the sand mining company and an overgrown sand track used by four-wheel drive vehicles. Trap stations are shown as solid dots together with the position of a belt transect (three trap stations wide) rising 4 m from the swamp and wet heath on the left up the vegetated old dune to dry heath and woodland on the right. This figure is adapted from Fox (1981, 1982).
Results and discussion
Topography on the Big Gibber study site rises 4 m from swamp and short wet heath up the vegetated old dune to tree heath (or woodland) and a belt transect along this moisture gradient was used to assess how small mammals used these trap stations (see Figure 2). Using Specht’s (1970) classification of vegetation, seven vegetation types (macrohabitats) were identified and mapped on the Big Gibber study site (see Fox 1981; Fox and Fox 1981). Six of these vegetation types were found on the belt transect shown in Figure 2, and in order from wettest to driest they are: swamp; short wet heath; tall wet heath; short dry heath; tall dry heath; tree-heath. These terms will be used in the remainder of this paper.
Eight species of ground-dwelling small mammals were commonly trapped on the Big Gibber site. Although the peramelid marsupial Isoodon macrourus (northern brown bandicoot) was present, the adults were too large to be regularly caught in the collapsible live traps used. However, they did not unduly impact the availability of traps for other species. Consequently, bandicoot captures have not been included in any of the analyses. Each of the seven remaining species comprised more than 5 % of the total captures and all were included in the analyses. These species were: the native murid rodents Rattus lutreolus (swamp rat), R. fuscipes (bush rat), Pseudomys novaehollandiae (New Holland mouse), and P. gracilicaudatus (eastern chestnut mouse); the introduced murid rodent Mus musculus (house mouse); together with the dasyurid marsupials Antechinus stuartii (brown antechinus) and Sminthopsis murina (common dunnart).
Distribution of species along this habitat gradient. Figure 3 illustrates how the four most abundant small mammal species were distributed along this single moisture gradient (habitat resource) before the August 1974 wildfire. The habitats range from swamp and wet heath at the wet end to dry heath and woodland at the dry end, and the graph uses data derived from Fox (1981). The four most abundant small mammal species show a replacement sequence as the moisture levels change along the transect. Each species appears to select habitat according to which type best meets its requirements, but this needs to be tested (see below), although where the house mouse was captured was more than likely to be determined by interactions with the other species. Significant interactions have already been well demonstrated for these species with replicated, controlled removal experiments (see Fox and Pople 1984; Fox and Gullick 1989; Higgs and Fox 1993; Thompson and Fox 1993; Luo and Fox 1995; 1996; Fox and Luo 1996; Luo et al. 1998; Morris et al. 2000).
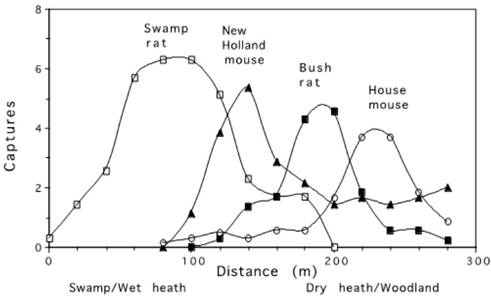
Figure 3 Distribution of the four most abundant species of small mammals, Swamp rat, (Rattus lutreolus) New Holland mouse (Pseudomys novaehollandiae), bush rat (Rattus fuscipes) and house mouse (Mus musculus), along a moisture gradient from wet to dry habitats (in terms of number of captures in May, June and July 1974, before the August 1974 wildfire). Captures along a belt transect (three trap stations wide, shown on Figure 2) were used with a three-point weighted average smoothing (1:2:1) applied to the raw data. Figure adapted from Fox (1981), with full methodology that was used and maps of habitat patches.
Post-fire succession brings a temporal scale to when each species may occupy a site. Five years of post-fire trapping results for the Big Gibber site are shown in Figure 4, with wet habitats shown in the upper panel and dry habitats shown in the lower panel for greater clarity and simplicity. There were only three individuals of Pseudomys gracilicaudatus (eastern chestnut mouse, early successional species) on the long-unburned Big Gibber site before the fire (hence they were not included on Figure 3). However, their abundance increased in the post-fire period as shown in Figure 4.

Figure 4 The succession for small mammals regenerating post-fire. The upper panel shows species from wet habitats, Pseudomys gracilicaudatus (eastern chestnut mouse), Rattus lutreolus (swamp rat) and Antechinus stuartii (brown antechinus). The lower panel shows species from dry habitats, Pseudomys novaehollandiae (New Holland mouse), Rattus fuscipes (bush rat) and Sminthopsis murina (common dunnart). Relative abundance of each species is shown as the proportion of the maximum abundance reached by that species. Values for each species before the fire are shown adjacent to the left axis. The house mouse (Mus musculus) appeared in both wet and dry habitats, but at different times in the succession. Figure is adapted from Fox (1982).
Testing for habitat selection versus habitat use. Do these species in fact select habitat, or are they relegated to suboptimal habitat by a dominance hierarchy, or do they merely use habitat in the same proportions in which it occurs? This has been tested using the two species of rodents that were most commonly captured in wet habitats (swamp rat and chestnut mouse) during the post-fire period. This test included all trap stations over the entire study grid, not just the trap stations from the transect shown in Figure 2. Both species showed a much higher proportional use of the wet habitats and a much lower proportional use of the dry habitats (see Figure 5) when measured against the availability of habitats over the entire study grid (i. e., the number of trap stations in each habitat type). The percentage use of wet habitats varied from 35 to 82 % whereas for dry habitats it ranged from 0 to 20 %. In this analysis the macrohabitats were defined using cluster analysis (for detailed methods used see Haering and Fox 1995) and are similar but not identical with those used in Fox (1981, 1982), although the differences are only slight in terms of where the habitat boundaries were drawn. While both species overlapped substantially in habitat requirements, they have been demonstrated to exhibit significant habitat partitioning when measured at the scale of the local trapping grid (Haering and Fox 1995).

Figure 5 Macrohabitat use by each species shows that both the eastern chestnut mouse (Pseudomys gracilicaudatus) and the swamp rat (Rattus lutreolus) choose or select wet habitats and avoid dry habitats, in relation to the number of stations of each habitat type available. The percentage of trap stations used by each species in each habitat is given on top of each bar (data from Haering and Fox 1995).
For each species, the frequency distribution of where they were captured across the six habitats differed significantly from the availability of trap stations in those habitats (P. gracilicaudatus, X2 = 26.8, P < 0.005, 5 d.f.; R. lutreolus X2 = 29.3, P < 0.005, 5 d.f.). Both species clearly most commonly occupied macrohabitats 1 to 3 (wetter heath) in comparison with much reduced occupation of macrohabitats 4 to 6 (drier heath). Captures at stations from each of the first three macrohabitats (see Haering and Fox 1995) provide a separate analysis, as they are the more used habitats. The presence or absence of each species from each trap station and the availability of trap stations in each macrohabitat provide the marginal totals for a X2 test. The distribution of captures for P. gracilicaudatus did indeed differ significantly from availability in habitats (X2 = 8.96, P < 0.05, 2 d.f.), showing strong positive selection of macrohabitat 1 (short dense wet heath) and choosing macrohabitat 3 less than expected, whereas macrohabitat 2 was not chosen significantly more than its availability would indicate. The distribution of captures in these three habitats for R. lutreolus did not differ significantly from the habitat availability (X2 = 1.87, P > 0.5, 2 d.f.).
Longer-term succession in wet heath habitats. Here, I concentrate upon the wet heath habitats occupied by chestnut mice and swamp rats to take a closer look at the impact of wildfire on this part of the small mammal community (see Figure 6). A similar case has been well supported for the dry heath habitats (see Haering and Fox 1997) and has been well documented already in analyses of long-term study sites (see Fox 1996). The Big Gibber study site had been monitored before the August 1974 wildfire so that an estimate of population density for each species before the wildfire was available. This site had two such fires (August 1974 and August 1980) in the time it was monitored (1974 to 1996) and analyses of the long-term results of this and other related studies has been published (Fox 1996); for full details of the site and methods used see Fox (1982). In both fire cycles there was a continuing replacement of the chestnut mouse by the swamp rat with increasing time since fire.
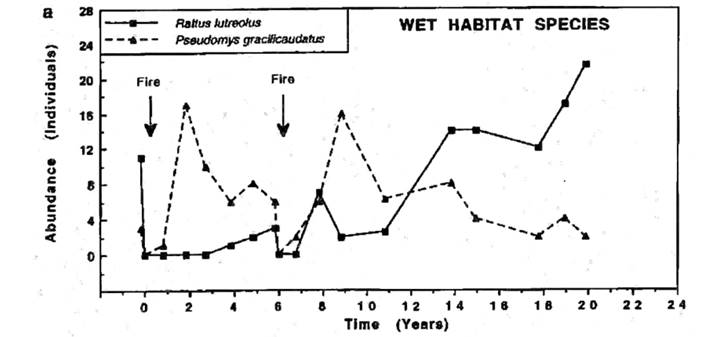
Figure 6 Number of Rattus lutreolus (swamp rat) and Pseudomys gracilicaudatus (eastern chestnut mouse) individuals captured on the 7-ha Big Gibber study site following wildfires in August 1974 and August 1980 with zero time set to the time of the first fire. This figure is adapted from Fox (1996).
Do these animals respond to changes in the vegetation succession rather than to time per se? This has been demonstrated conclusively on different sites with similar wet heath macrohabitat by Monamy and Fox (2000) using microhabitat measurements of horizontal vegetation density in different layers, most significantly for the layer 0 to 20 cm from the ground level. Two high-intensity wildfires 20 years apart at two different sites, but with very similar wet heath vegetation, produced very different patterns of succession when measured against time since fire. The early seral stage specialist P. gracilicaudatus (eastern chestnut mouse) appeared after one year at the first site but appeared after only three months at the second site. The late seral stage specialist R. lutreolus (swamp rat) took 3.6 years to recolonize at the first site but appeared after only four months at the second site. However, when these responses were graphed not against time, but against a vegetation recovery index (a measure of how rapidly vegetation returned), the trajectories for a species following each of the two fires were concurrent for that species, although for each species the trajectory was different (see figures 4 and 5 in Monamy and Fox 2000:584). The vegetation recovery index was derived from horizontal vegetation density measurements made for different layers of vegetation, from ground level to 2 m.
The role interspecific competition plays in small mammal succession. Does interspecific competition play any role in this species replacement, in relation to the expression of habitat selection that was demonstrated in Figure 5? Similarly, does interspecific competition play any role in relation to regeneration time for this succession? Although graphs of the abundance of species are generally presented on a time scale, these graphs of the abundance of species would be better represented on a scale reflecting changes in the vegetation density as indicated above (see also Monamy and Fox 2000). Experimental analyses of these questions were carried out using replicated, removal experiments (see Higgs and Fox 1993). Figure 7 represents a summary of the results of an experimental removal of swamp rats from five 0.5-ha plots, each with 12 trap stations, in wet heath macrohabitat, when compared to five 0.5-ha control plots in adjacent similar habitat. On all plots each trap station was trapped for three nights in each trapping session. Two pre-removal trapping sessions (23 to 25 January and 8 to 10 February 1989) were undertaken to measure initial mean abundance, then five three-night trapping sessions were conducted (between 13 February and 11 March) during which all swamp rats captured on experimental plots were removed. Post-removal trapping sessions (five) were conducted between 22 March and 30 August 1989. This study concluded that there was very significant interspecific competition from the dominant swamp rat upon the chestnut mouse as seen in Figure 7. The reciprocal removal experiments (Thompson and Fox 1993) had already demonstrated how interspecific competition played an important role in these wet heath habitats. In summer, removal of the chestnut mouse demonstrated a significant competitive release in the abundance of the swamp rat, but in winter (by which time juvenile swamp rats had grown into adults) no significant competitive release was observed. “The different outcomes of such competition are determined by the size class structure of the larger species, thus producing seasonally variable interspecific competition” (Thompson and Fox 1993:264).

Figure 7 Mean standardized abundance (± SE) for the eastern chestnut mouse during each trapping session, using mean data for five replicates where swamp rats were removed from experimental plots for trap sessions 3 to 7. Abundance is shown as the departure from zero where zero represents the mean pre-removal abundance. Asterisks denote trapping sessions where both the Mann-Whitney U-test and Student's t-test demonstrate significant differences between control and experimental treatments (P<0.05). This graph is based upon data published by Higgs and Fox (1993).
An experimental vegetation clipping project (Fox et al. 2003) carried out on wet heath habitats in 1993 demonstrated conclusively that physically removing 60 to 70 % of the vegetation significantly reduced the abundance of R. lutreolus (swamp rat), whereas the abundance of P. gracilicaudatus (eastern chestnut mouse) remained relatively unchanged, thus producing a retrogression of the small mammal succession and demonstrating that the change in vegetation density was causal. This outcome clearly linked small mammal succession to vegetation density, instead of just successional time, by experimentation, in contrast to the observational link reported by Monamy and Fox (2000). A further experimental clipping project (Monamy and Fox 2010) carried out on plots adjacent to each of these same plots in wet heath habitats in 1994 physically removed 85 % of the vegetation. This experiment produced an even greater retrogression of the small mammal succession, with significant reductions in the abundance of both R. lutreolus (swamp rat) and P. gracilicaudatus (eastern chestnut mouse). The dry habitat species P. novaehollandiae (New Holland mouse) made an appearance, together with the more cosmopolitan M. musculus (house mouse), on plots where vegetation density had been made similar to that for vegetation supporting an early seral stage of this small mammal succession.
Experimental support for the habitat accommodation model. The vegetation removal experiments of Fox et al. (2003) and Monamy and Fox (2010) that produced a retrogression of the small mammal succession clearly provide experimental support for the habitat accommodation model, first proposed in Fox (1982) that followed from the earlier work of Connell and Slatyer (1977), which described both habitat facilitation and habitat tolerance models. The habitat accommodation model incorporates elements of both the habitat facilitation and tolerance models; small mammal species do not modify local physical conditions, as these are changing in response to external factors (vegetation regeneration following wildfire). Small mammal species enter the post-fire succession when the changing local physical conditions (regenerating vegetation) first meet their specific requirements (habitat facilitation model). This has been experimentally demonstrated with transplantation of P. novaehollandiae (New Holland mouse) onto differently aged early seral stages of vegetation regeneration following sand mining. These introductions showed that this species was not able to colonize sites that had been regenerating for less than 4.9 years (see Fox and Twigg 1991:281). However, when vegetation conditions move out of the optimal range for the small mammal species, the animals leave or are reduced in numbers (habitat tolerance model). The reductions in abundance may be caused by these species no longer being able to obtain an adequate share of the resources they need, as their competitive ability becomes reduced. The significant impact of interspecific competition for small mammal species in this succession has been demonstrated experimentally for wet heath species with replicated, reciprocal, removal experiments (Higgs and Fox 1993; Thompson and Fox 1993). The significant impact of interspecific competition for dry heath small mammal species in this succession has also been demonstrated experimentally (Fox and Pople 1984; Fox and Gullick 1989). The habitat accommodation model accounts for all species in this Big Gibber post-fire succession, as well as small mammal successions following sand mining (Fox and Fox 1978, 1984; Fox 1990 a, b; Fox 1996).
Even more support for this model was demonstrated when longer time scales for post-fire succession became available. For the Big Gibber site supporting both wet and dry heath habitats, the common dunnart (Sminthopsis murina) was not captured during the last three years of 14 years of post-fire trapping (see Fox 1996). Local extinction was confirmed with an even longer period of post-fire trapping on that same site, as noted by Fox and Fox (2000:30) “… with Sminthopsis murina now locally extinct for 6 years, although it has been captured within 3 km (those sites support an earlier successional stage). The eastern chestnut mouse (Pseudomys gracilicaudatus) has also now been absent for 2 years (Figure 5).”
The replacement sequence in small mammal succession. Here, I have examined the post-fire succession in coastal heathland, however, elsewhere (Fox and Fox 1978) we described a small mammal succession in similar coastal heathland regenerating following sand mining. Similar successions for open eucalypt forest near Seal Rocks in MLNP were described following disturbances by wildfire (see Fox and McKay 1981) and sand mining (see Fox and Fox 1984).
The more extensive succession demonstrated for this open forest post-fire, with species reaching their peak abundances before they were replaced, follow the order: house mouse (Mus musculus), New Holland mouse (Pseudomys novaehollandiae), common dunnart (Sminthopsis murina), brown antechinus (Antechinus stuartii), bush rat (Rattus fuscipes), as illustrated in Figure 8 (adapted from Fox 1996). In areas remaining undisturbed for very long periods, even the late successional bush rat decreased in abundance as the understory vegetation began to senesce. This senescence meant the vegetation became more open at ground level, which allowed an increased amount of light to reach the ground beneath the understory vegetation. Hence, vegetation succession became reinvigorated, as dormant seeds from early seral stage plants germinated and appear again in the understory. The mammalian succession also began again, when early successional species (house mouse, New Holland mouse) reappeared in the community at the same time that bush rat abundance had decreased (see Fox 1996:478 Figure 2). The habitat accommodation model for animal succession (Fox 1982; Fox 1990a) recognizes that animals enter the succession when their specific habitat requirements are met, and that they lose dominance in the succession through competitive interactions when species entering the succession outcompete them. One conclusion to be reached from this, relevant to habitat regeneration, is recognition that the species that can occupy any site will be largely determined by the habitat variables at that site, and that there are both spatial and temporal scales to these habitat variables.

Figure 8 An illustration of the small mammal succession following wildfire in open eucalypt forest near Seal Rocks in MLNP, using composite data from 1982-1994 taken from Fox (1996). Species shown are house mouse (Mus musculus), New Holland mouse (Pseudomys novaehollandiae), common dunnart (Sminthopsis murina), brown antechinus (Antechinus stuartii) and bush rat (Rattus fuscipes). Abundance (as individuals captured on each 1 ha trapping grid) is shown as a function of the time since last fire in years.
This same eucalypt open forest has also been subjected to sand mining (Fox and Fox 1984; Twigg et al. 1989), and it is possible to illustrate a similar small mammal succession for the early successional species (see Fox 1996). Some of these data (from 1982, 1987, 1992) have been redrawn in Figure 9 and show three species entering the succession in the order: house mouse, New Holland mouse, common dunnart. This pattern reflects the post-fire succession, but on a more drawn-out time scale as the vegetation regeneration proceeds much more slowly after sand mining than after fire. Later trapping in 1997 revealed the presence of brown antechinus and bush rat for the first time on these regenerating sand-mined sites, which had been sampled every five years (1982, 1987, 1992, 1997). Because regeneration following sand mining is so much slower than regeneration following wildfire, it is only the early part of small mammal post-fire succession that has yet been observed on any regenerating sand mining path, even though these have been trapped for more than fifteen years on sites that have been regenerating for up to 25 years. The similarity with the post-fire succession is strong and is in agreement with the comparisons made for vegetation succession reported from three types of disturbance (fire, clearing, and mining) in similar forest/woodland habitat at Tomago, near Newcastle in NSW (Fox et al. 1996). Factors influencing plant species richness on these same sites at Tomago have been studied in detail (Ross et al. 2002), while the interaction of multiple disturbance types has also been shown to play an important role in regenerating and rehabilitating areas (Ross et al. 2004).
How various factors can have influence upon parameters such as mammal species richness at any one spot has been examined (Fox and Fox 2000). Behavioral interactions between species have also been shown to play an important role in determining how species may co-occur at any one place (see Righetti et al. 2000). As well as considering species richness, however, it is also necessary to consider which combination of small mammal species might be found assembled into any one community at any one spot in the landscape.
Guild assembly rules. Taking these ideas and applying them to the data accrued from my years of field work in MLNP (Fox 1980, 1981, 1982, 1985) led me to propose what would become a guild assembly rule (Fox 1987) for the small mammals that had been encountered at any one site. This assembly rule was first stated this way: “There is a much higher probability that each species entering a community will be drawn from a different functional group (genus or other taxonomically related group of species with similar diets) until each group is represented, before the cycle repeats” (Fox 1987:201).
My guild assembly rule was developed in an empirical manner from my observations of the structure of small mammal communities occupying different patches of habitat (see Fox 1980, 1981, 1987) but lacked any theoretical derivation. However, in a later publication (Morris and Knight 1996), Doug Morris demonstrated this guild assembly rule was a probabilistic consequence of adding guild structure to MacArthur-Tilman models of consumer resource competition (Tilman 1982). This provided the theoretical underpinning for my empirically derived rule (Fox 1987). I included this theoretical derivation when I dealt with the genesis and full development of this guild assembly rule (Fox 1999). Later, Doug Morris was able to come from Canada and visit my study sites to test out his models on these same wet heath habitats in MLNP (see Morris et al. 2000). This guild assembly rule has been applied to a wide range of species and landscapes including shrews in northeastern USA (Fox and Kirkland 1992), rodents from multiple sites in Valdivian rainforests in southern Chile, South America (Kelt et al. 1995), lemurs from Madagascar (Ganzhorn 1997) and desert rodent assemblages from North America and Asia (Kelt et al. 1999), all far distant from, and quite different to the coastal heathlands and eucalypt forests in eastern Australia where this rule had been empirically derived. A stringent test of this rule was reported when I applied it from data that Jim Brown had compiled for seed-eating rodents from the species-rich southwestern deserts of North America (Fox and Brown 1993). That data set came from 202 sites, widely distributed over an area of approximately 640,000 km2, including all of the Chihuahuan, Sonoran, Mojave, and Great Basin Deserts north of the U.S.-Mexican border (see Brown and Kurzius 1987), where interspecific competition had already been confirmed from a long-term experimental study (Heske et al. 1994). Further analyses of this guild assembly rule have also been undertaken in these southwestern deserts (see Brown et al. 2002 and references therein).
Factors such as habitat diversity, disturbance, species interactions, and guild assembly rules have all been shown to influence mammal species richness at any one site (Fox and Fox 2000). These factors and others have also been examined as linkages to landscape ecology and responses to climate change (see Hilty et al. 2019).

Figure 9 The small mammal succession following sand mining along Bridge Hill Ridge, through eucalypt open forest, similar to forest near Seal Rocks MLNP shown in Figure 8, using composite data taken from Fox (1996) for trapping periods in three separate years, 1982, 1987, 1992. Abundance (as individuals captured on each 1 ha trapping grid) is shown as a function of regeneration age in years, for house mouse (Mus musculus), New Holland mouse (Pseudomys novaehollandiae), and common dunnart (Sminthopsis murina).
From the information contained in the studies reviewed and synthesized here, I conclude that:
Small mammal species do actively choose or select, rather than just use, habitat along a moisture gradient ranging from swamp through dry heath into tree heath (or woodland);
Small mammal species display a sequential replacement series as part of the successions observed in both heathland and eucalypt open forest habitats following disturbance by wildfire and sand mining;
While the small mammal successions that follow wildfire and sand mining are very similar, the latter occurs much more slowly than post-fire succession, reflecting slower vegetative succession after sand mining relative to wildfire;
Replicated and controlled removal experiments have shown that interspecific competition plays a very important role in these small mammal successions and that body size is an important factor in this interspecific competition;
Experimental support has been demonstrated for the habitat accommodation model in small mammal successions following disturbances by wildfire and by sand mining;
A small mammal guild assembly rule that operates in these communities (as well as in a diverse range of landscapes on different continents) is a probabilistic consequence of adding guild structure to MacArthur-Tilman models of consumer resource competition.
All of these can be major influences upon the community and landscape ecology of small mammals. The particular combination of small mammal species that may be observed to occur at any one place can be influenced by a suite of factors that vary spatially and temporally.