Human-induced climate change will lead to higher temperature and lower rainfall in several regions of the world, and this can threaten the persistence of forest ecosystems (Hanson & Weltzin 2000, Walck et al. 2011). Seasonally dry forests are particularly sensitive to these changes because seed germination and the early development of tree seedlings are constrained to the rainy season (Vieira & Scariot 2006). In this way, the climatic changes that are expected to occur in this period of the year can strongly jeopardize tree recruitment in these ecosystems.
Climate change can also impair forest recovery in human-disturbed areas. Seasonally dry forests originally covered more than 40 % of the American continent, but more than half of these ecosystems were converted in agricultural and grazing fields over the past four centuries (Vieira & Scariot 2006). Several of these fields are currently abandoned because of their low productivity and, although this could be perceived as an opportunity for the natural recovery of forested areas, tree seedling establishment in these habitats is constrained by elevated temperatures and low water availability (Badano et al. 2015, González-Salvatierra et al. 2013). Thus, forest recovery in abandoned fields could be even harder to achieve in the future because of the advance of climate change.
The persistence and recovery of seasonally dry forests under climate change will then partially rely on the ability of tree seeds and young seedlings to cope with warmer and drier conditions during the growing season (i.e., the rainy season). As several tree species from these ecosystems produce recalcitrant seeds (Zavala-Chávez 2001, Kettle 2012), these environmental changes may reduce seedling emergence because they may enhance seed water loss and promote the death of their embryos (Walck et al. 2011). Thus, seedling emergence in climate change scenarios will depend on whether seeds can remain viable until favourable conditions to germinate occur, which will also depend on whether the rainfalls they receive are enough to trigger germination. The early development of the resulting seedlings, on the other hand, will depend to their capability to display functional responses addressed to prioritize survival over other plant processes. The most common responses of tree seedlings exposed to elevated temperatures and/or water deficits are slower growth rates (Valladares & Sánchez-Gómez 2006), accelerated foliar phenology (Fu et al. 2013), smaller leaf areas (Quero et al. 2006), lower water contents (Gond et al. 1999, Aranda et al. 2005) and reduced light harvesting for photosynthesis (Xu & Zhou 2006). These functional responses could allow tree seedlings to survive under climate change conditions, but the elevated investment of resources that they should perform to maintain and replace vegetative structures may ultimately impair their development.
Studies dealing with the potential consequences of climate change on forest ecosystems have been mainly focused on assessing how this phenomenon will affect survival and growth of adult trees, or in modelling the future distribution of tree species (e.g., Engelbrecht et al. 2007, Gómez-Mendoza & Arriaga 2009, Malhi et al. 2009). Some authors have also experimentally evaluated how climate change will affect tree recruitment in the field. However, these studies have mostly evaluated the effects of warming in high-latitude or alpine forests (e.g., Batllori et al. 2009, Piper et al. 2013) or the effects of increasing drought in Mediterranean forests (e.g., Lloret et al. 2004, Ramírez-Valiente et al. 2009). In this way, the combined effects that increasing temperature and decreasing rainfall may have on the initial development of tree seedlings in seasonally dry forests have received less attention. These forests, however, harbour an elevated number of endemic trees whose distributions are determined by specific regimens of temperature and rainfall (Pennington et al. 2009). Thus, tree species from these ecosystems could be highly prone to extinction if increasing warming and drought constrain the initial stages of their recruitment processes.
In this study, we evaluated the emergence, survival and growth of seedling of an oak endemic to seasonally dry forests of Mexico when exposed to climate change conditions during its recruitment season. The target species was Quercus eduardii Trel. (Fagaceae, section Lobatae). This is a conspicuous tree within these forests but, unlike most other oaks from these ecosystems, this species is also able to recruit in deforested areas (Alfonso-Corrado et al. 2007). This makes Q. eduardii a promising candidate to assess how climate change will affect the early recruitment stages of trees in both, forests and man-induced clearings. Our overall expectation was that all plant processes mentioned above will be impaired by the combined effects of increased temperature and reduced rainfall during the growing season. Further, surviving seedlings subjected to climate change conditions were also expected to display functional responses matching with increased thermal and/or water stress. Indeed, as oaks usually prefer shaded understory habitats to recruit (Broncano et al. 1998, Li & Ma 2003, Puerta-Piñero et al. 2007, Gómez-Aparicio et al. 2008), we also hypothesized that all these effects should be deeper in man-induced clearings than at the interior of forests.
Materials and methods
Study site. This study was conducted at National Park “El Potosí”, located in the westernmost section of Sierra Madre Oriental, state of San Luis Potosí, Mexico. The substrate across the study region is composed by leptosols (highly calcareous, shallow and stony soils) that reach maximum depths of 10-15 cm to the bedrock. Climate is temperate, with mean air temperature of 20 °C and annual precipitation of 620 mm (Fernández-Eguiarte et al. 2012). Up to 80 % of rainfalls occur are concentrated between July and November, but sparse rainfalls can occur until February. After this period, there is a marked dry season (Fernández-Eguiarte et al. 2012). The original vegetation of this region was composed by mixed oak forests of Quercus mexicana, Quercus resinosa, Quercus viminea and Quercus eduardii, together with a few trees of the genus Pinus (Zavala-Chávez & García-Sánchez 1999). Local inhabitants induced several clearings in the forest for cattle grazing, but most of these sites are currently abandoned because of the intensive soil erosion they were subjected because of wind and water runoff after vegetation was removed.
Target species. Sparse saplings of Q. eduardii can be found in the clearings of the study site (less than 5 individual/ha), while no other tree species is detected in these open areas. This oak is a perennial tree that produces acorns (single-seeded, nut-type fruits) in spring and release them in late summer (October-September). As occurs in most oak species, the acorns of Q. eduardii are extremely recalcitrant and quickly loss viability if their water contents drop below 25-30 %, and germination only occurs after acorn water contents surpass 80 % (Zavala-Chávez & García-Moya 1996). For this reason, seedling emergence in the field is usually detected after intense rainfalls (Zavala-Chávez 2001). In the study region, emergence of oak seedlings occurs between October and December (second half of the rainy season) and their early development just extends until February because plant growth in these ecosystems stops at the beginning of the dry season (Zavala-Chávez & García-Sánchez 1999).
Climate change predictions. We used the geodatabases of Fernández-Eguiarte et al. (2012, 2014) to estimate the changes in temperature and rainfall expected to occur in study site during the oak growing season (October-February). These geodatabases have a resolution of 1 km2 per pixel and current values of both variables are monthly averages that integrate climatic data from 1902 to 2011 (Fernández-Eguiarte et al. 2012). Future values are monthly predictions for 2040 according to the HadGEM2-ES general circulation model under the four representative concentration pathways proposed by IPCC (RCP2.6, RCP4.5, RCP6.0 and RCP8.5) (Fernández-Eguiarte et al. 2014). These estimates indicated that air temperature by 2040 will increase 1.2-1.7 °C between October and February, while rainfalls will decrease 10-17 % (Table 1).
Table 1 Net increases in air temperature and percent decrease in rainfall predicted to occur by 2040 across the study site during the growing season of oaks (October-February). These differences are provided for the four representative concentration pathways proposed by IPCC (RCP2.6, RCP4.5, RCP6.0 and RCP8.5) considering the current climate as reference.
Climate change scenario | Air temperature | Rainfall |
---|---|---|
RCP2.6 | +1.7 °C | -15 % |
RCP4.5 | +1.2 °C | -10 % |
RCP6.0 | +1.4 °C | -12 % |
RCP8.5 | +1.4 °C | -17 % |
Experimental design and plant material. Our field experiment was performed during the oak growing season comprised between October 2015 and February 2016. For this, in the study site we selected a well-preserved primary oak forest (21° 57’ 08’’ N, 100° 21’ 01’’ W, 1658 m elevation) and a nearby man-made clearing (21° 57’ 15’’ N, 100° 21’ 02’’ W, 1665 m elevation). Canopy cover in the forest site was 87 % and, across the growing season 2015-2016, the photosynthetic photon flux density (PPFD) in the understory averaged 371 µmol m-2 s-1 during daytime (07:00 to 19:00 h). The clearing has scarce vegetation cover and PPFD during daytime averaged 1053 µmol m-2 s-1 at this habitat. In both habitats, PPFD was assessed with automatized dataloggers (HOBO Pendant-UA-002, Onset Computer Corporation, Bourne, USA) programmed to measure this variable every hour.
At each habitat, we selected ten point-sites and removed leaf litter and rocks in a radius of 3 m. The half of these sites were randomly assigned to control plots that remained under the current environmental conditions. The other half of these sites were assigned to climate change simulation plots (hereafter, CCS) in which we increased temperature and reduced rainfall. Temperature increases in CCS plots were induced with hexagonal open-top chambers (Marion et al. 1997) of transparent acrylic (3 mm thick, wavelength transmission 280-750 nm). This design (Figure 1) reduces wind speed and passively increases air temperature by 1-2 °C relative to the external environment, without causing large changes in light conditions within the chambers (Aragón-Gastélum et al. 2014). To reduce rainfall in CCS plots, we used rainout shelters constructed with U-shaped channels of transparent polycarbonate (1.5 mm thick, 10 cm wide, 3 m length) supported on metallic frames (Yahdjian & Sala 2002). As the amount of rainfall intercepted by these structures depends on the number of channels disposed on the frames, before starting the experiment (July-August 2015) we conducted a series of field trials to determine how many channels were required to reduce precipitation to the desired levels (i.e., 10-20 %). These tests were conducted in forest clearings because there are no predictions of climate change to understory habitats. Field trials indicated that four U-shaped channels located 25-30 cm above the open-top of chambers reduce precipitation by 14-19 % (Figure 1) and, thus, we used this design. Control plots, on the other hand, were fenced with wire mesh (13 mm openness) to prevent the access of acorn and seedling predators that may interfere with the experiment (Figure 1).
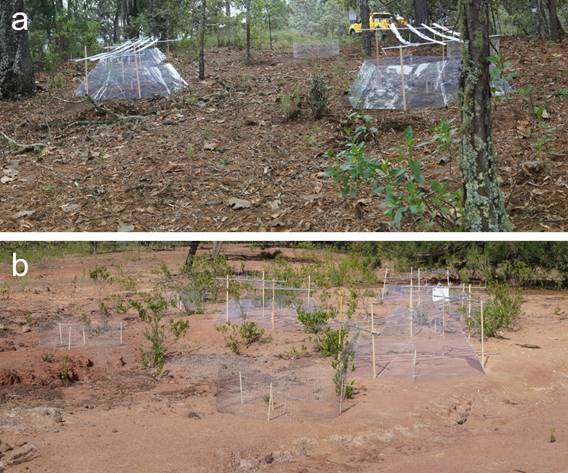
Figure 1 Experimental units used to assess effects of climate change on the recruitment of Quercus eduardii in the forest site (a) and in the clearing (b). The plates show control plots fenced with wire mesh and the climate change simulation plots (CCS plots). Each CCS plot consisted in an open-top chamber of transparent acrylic and a rainout shelter of transparent polycarbonate.
Experimental plots were established in the field on October 09, 2015 and, at this time, we conducted extensive searches of Q. eduardii individuals with recently released acorns. This resulted in 23 reproductive trees and 100 mature acorns were collected beneath the canopy of each of them. Because acorns collected in the field may be unviable due to seed desiccation or infestation by insects (Ramos-Palacios et al. 2014), we used the float test to separate viable and unviable acorns (Gribko & Jones 1995). For this, acorn cupules were removed, and nuts were placed in water during 2 h. After that, sunken and floating acorns were assumed as viable and unviable, respectively (Gribko & Jones 1995). This resulted in 951 viable acorns (59 % of the acorns were not viable) that were pooled in a single sample and stored in a refrigerated chamber (5 °C) to prevent water loss and germination.
On 14 October 2015, we randomly selected 400 viable acorns and grouped them in 20 batches (20 acorns per batch). These batches were placed in water during 48 h to fully hydrate acorns, as they require to be near to their maximum hydration capacity to germinate (Zavala-Chávez & García-Moya 1996, Zavala-Chávez 2001). On 16 October 2015, these acorns were taken to the field and randomly assigned to controls and CCS plots located in both, the forest and the clearing. In the experimental plots, acorns were sowed 2-cm depth to emulate the hoarding behaviour of field mice, which are their main secondary dispersers in this region (Ramos-Palacios et al. 2014). Sowing positions were marked with small wood stakes to facilitate the monitoring of emerged seedlings. Seedling emergence and survival were monitored every seven days until 26 February 2016 (19 weeks), which approximates to the end of the oak growing season. At the end of the experiment, ungerminated acorns were recovered and taken to the laboratory to assess their viability. For this, we performed a small incision on the pericarp of recovered acorns and incubated them in solution of 1 % trazolium salt (2,3,5-triphenyltetrazolium chloride) during 24 h in dark. This test relies on the reaction of the colourless tetrazolium salt with hydrogen released by active dehydrogenase enzymes, which is only possible if seed embryos are alive. This reduces the tetrazolium salt to formazan, a non-diffusible red dye that stains the embryos if seeds are viable (Bewley et al. 2013).
Microclimate monitoring. To assess the magnitude of the environmental changes induced within CCS plots with open-top chambers and rainout shelters, as compared to controls, we randomly selected three experimental units of each climate treatment in the forest and the clearing. At the centre of these plots, we fixed installed air temperature/relative humidity dataloggers (HOBO U23-Pro-V2, Onset Computer Corporation, Bourne, USA), which were fixed 20 cm above ground on wood stakes. In these plots we also measured soil temperature by burying temperature dataloggers at 5 cm depth (HOBO Pendant-UA-002, Onset Computer Corporation, USA). Dataloggers were programmed to record climatic variables every hour during the entire experiment. Further, to assess changes in rainfall, in these plots we installed automatized pluviometry sensors (HOBO S-RGB-M002, Onset Computer Corporation, USA) programmed to record precipitation at each rainfall event.
Besides these automatized measures, every week of the experiment we measured the soil volumetric water content in all experimental plots with a time-domain reflectometer probe (Field Scout TDR 300, Spectrum Technologies, USA). Soil water contents were measured at 3.8 cm depth, corresponding to the acorn germination environment, and at 7.6 cm deep, corresponding to the environment of seedling roots. Deeper measures were not performed because soils of this region are too shallow (10 cm to the bedrock). At each week, we took six measurements of soil water content on random points within each experimental plot and these values were automatically averaged by the device. We did it to deal with the potential microvariability is soil water content within each plot.
Seedling responses. Besides assessing seedling emergence and survival, at each monitoring date we measured the height of all surviving seedlings using digital callipers (CALDI-6MP, Truper, Mexico). At each week we also recorded unfolding and senescence of the first leaf on each seedling. For this, we marked the petioles of recently emerged leaves with a small drop of permanent white paint and foliar senescence was assumed to start when leaves became yellowish-brownish coloured. We did not record phenology on further leaves because Q. eduardii is an evergreen species and this would have required experimental periods beyond the oak growing season.
At the end of the experiment, at each experimental plot we selected three surviving seedlings with at least three mature leaves (other than the first leaf because it was used to assess foliar senescence). On these leaves we measured photosynthetic photon flux density (PPFD), foliar temperature (FT) and effective quantum yield of photosystem II (ɸ PSII ) with a portable pulse-modulated chlorophyll fluorometer (MINI-PAM II, Heinz Walz, Germany). Values of ɸ PSII were estimated as (F m - F t ) / F m , where F t is leaf fluorescence under steady-state illumination (i.e., environmental levels of light) and F m is the maximum fluorescence when a saturating pulse of actinic light (≈ 2,500 µmol photon m-2 s-1, in our case) is superimposed on environmental illumination (Maxwell & Johnson 2000). These measures were conducted on sunny days (27 February 2016 within the forest; 28 February 2016 in the clearing) between 10:00 and 12:00 h. These leaves were later harvested, stored in hermetic plastic bags placed in ice prevent excessive water loss and taken to the laboratory. Fresh biomass (F b ) of leaves was determined within three hours after harvesting using an analytical balance (Cubis, Sartorius, Germany). Leaves were later placed in botanical presses and dried in a ventilated stove at 60 °C until their dry biomass (D b ) remained constant (about 48 h). With this data, we estimated the percent water content (PWC) of leaves as (F b - D b ) / F b and this variable was used as surrogate of the water status of seedlings in the field (Gond et al. 1999). After that, leaves were scanned (Perfection V39 Scaner, Epson, Japan) and images were processed with MideBMP 4. 2 (Ordiales-Plaza 2000) to determine leaf areas (LA). These data were used to estimate the specific area (SLA) of leaves as LA / D b . Values of PPFD, FT, ɸ PSII , PWC and SLA were averaged across leaves of the same seedling to avoid pseudoreplication in the statistical analyses described below.
Statistical analysis. Two-way repeated measures ANOVAs were used to compare environmental variables between climate treatments (controls vs. CCS plots) and habitat types (forest vs. clearing). For these analyses, air temperature, soil temperature and air relative humidity recorded by each datalogger (n = 3 for each combination of climate treatment x habitat type) were averaged for every week of the experiment (repeated measures = 19 weeks). We also calculated the weekly accumulated precipitation recorded by each pluviometry sensor (n = 3 for each combination of climate treatment x habitat type) but, because ANOVAs do not admit groups with zero variance (Kutner et al. 2005), those weeks with no rainfalls were excluded from the analysis (repeated measures = 9 weeks, in this case). Soil water contents were separately compared for each soil depth (3.8 cm and 7.6 cm) and these analyses were conducted with the values obtained from all experimental units (n = 5 per climate treatment; repeated measures = 19 weeks).
Failure-time-analyses were used to compare seedling emergence rates among combinations of climate treatments and habitat types. In these analyses, acorns received values of 0 (zero) at the beginning of the experiment, which were turned into 1 (one) on the date in which shoot emergence was recorded. Ungerminated acorns retained the zero-value until the last monitoring date. With these binary data (0-1), we estimated standardized seedling emergence rates with Kaplan-Meier method and compared them with Gehan-Wilcoxon tests (Kleinbaum & Klein 2012). We used the same statistical procedures to compare seedling survival rates but, in this case, only emerged seedlings were included in the analyses (i.e., ungerminated acorns were not considered). In this case, acorns that gave rise to seedlings received a value of 1 (one) at the beginning of the experiment and this value was turned into 0 (zero) on the date in which the death of each seedling was recorded. Those seedlings that survived until the last monitoring date retained the one-value.
To determine how stem height increased with time, as well as to determine whether these relationships varied across combinations of climate treatments and habitat types, we used a multiple nonlinear regression with categorical variables (Kutner et al. 2005). This analysis only included seedlings that remained alive until the end of the experiment (63 seedlings in controls from the forest, 34 seedlings in CCS plots from the forest, 47 seedlings in controls from the clearing, 28 seedlings in CCS plots from the clearing). Because stem height stabilized over time, we used an asymptotic regression function of type H t = β 0 + (β 1 / T); here, H t is seedling height at the t th monitoring date, T is the number of days elapsed after shoot emergence (time), β 0 is the intercept of the regression curve and β 1 is the increase in seedling height per time unit. In this model, climate treatments (controls vs. CCS plots) and habitat types (forest vs. clearing) were included as categorical variables with two levels each, allowing all possible interactions among predictive variables. Thus, the analysis compared the parameters (β 0 and β 1) that define the shape of these relationships across combinations of climate treatments and habitat types.
Unfolding and senescence rates of the first leaf were compared among combinations of climate treatments and habitat types with failure-time-analyses similar to those described above. These analyses included all seedlings that survived until the end of the experiment. On the other hand, two-way ANOVAs and post-hoc Tukey tests were used to compare PPFD, FT, ɸ PSII , PWC and SLA. In these analyses, climate treatments and habitat types were included as predictive variables with two levels each.
Results
Climate manipulation. In both habitats, air and soil temperatures were significantly higher in CCS plots than in controls across the entire experiment (Table 2). This indicates that open-top chambers were effective for inducing warmer conditions and, as expected, these differences were larger in the clearing than within the forest. In the clearing, average air temperature in CCS plots was 1.7 °C higher than in controls, while this difference was 1.4 °C in forest (Figure 2A). Average soil temperature in the clearing was 2.5 °C higher in CCS plots than in controls, but this difference barely surpassed 1.9 °C within the forest (Figure 2B). Air relative humidity in both habitats was lower in CCS plots than in controls (Table 2) and these differences were also larger in the clearing than within the forest (Figure 2C).
Table 2 Results of repeated measures ANOVAs addressed to assess effects of climate treatments and habitat types on the environmental variables measured across the field experiment. The table shows the effects of the main factors (climate treatment = Ct; habitat types = Ht), the repeated measures (weeks of the experiment = W) and their interaction. In all cases, significant effects are indicated with an asterisk (critical α = 0.05).
Environmental variables | ||||||
---|---|---|---|---|---|---|
Sources of variability | Air temperature | Soil temperature | Precipitation | Air relative humidity |
Soil water content (3.8 cm depth) |
Soil water content (7.6 cm depth) |
Ct | F (1,8) = 967.870* | F (1,8) = 2253.548* | F (1,8) = 4226.253* | F (1,8) = 10503.120* | F(1,16) = 9855.451* | F (1,16) = 8174.111* |
Ht | F (1,8) = 813.624* | F (1,8) = 4766.193* | F (1,8) = 4977.6133* | F (1,8) = 3795.583* | F (1,16) = 11989.584* | F (1,16) = 7108.872* |
Interaction Ct-Ht | F (1,8) = 10.571* | F (1,8) = 36.443* | F (1,8) = 1.0800 | F (1,8) = 379.460* | F (1,16) = 1.775 | F (1,16) = 246.238* |
W | F (18,144) = 1652.206* | F (18,144) = 833.448* | F (8,64) = 60872.535* | F (18,144) = 36379.397* | F (1,16) = 22472.838* | F(1,16) = 12338.098* |
Interaction Ct-W | F (18,144) = 0.893 | F (18,144) = 0.828 | F (8,64) = 527.812* | F (18,144) = 34.439* | F (18,288) = 244.748* | F (18,288) = 313.880* |
Interaction Ht-W | F (18,144) = 9.815* | F (18,144) = 24.974* | F (8,64) = 419.790* | F (18,144) = 69.387* | F (18,288) = 620.472* | F (18,288) = 138.541* |
Interaction Ct-Ht-W | F (18,144) = 0.809 | F (18,144) = 0.6228 | F (8,64) = 1.819 | F (18,144) = 13.626* | F (18,288) = 37.133* | F(18,288) = 54.255* |
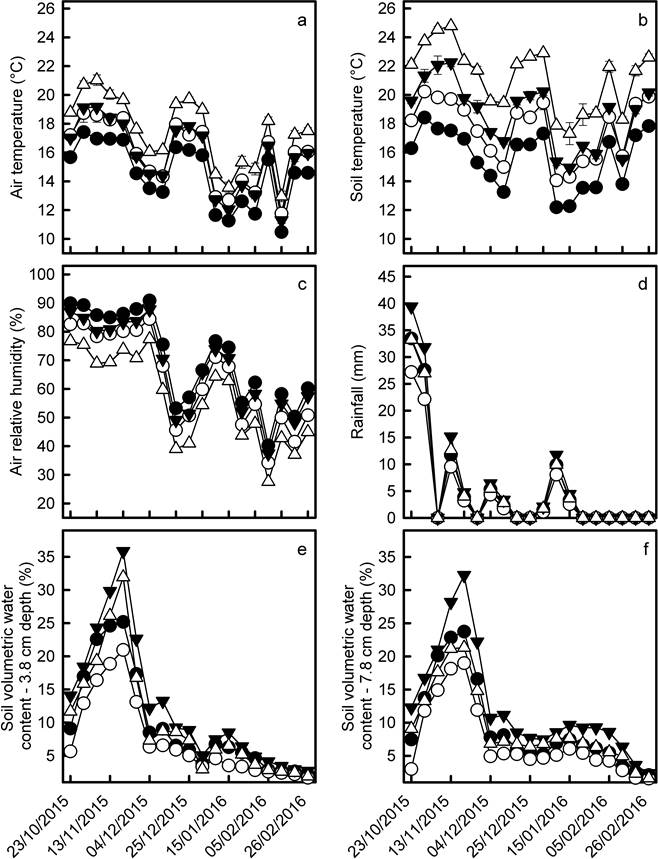
Figure 2 Average values (± 95 % IC) of air temperature (a), soil temperature (b), air relative humidity (c), rainfall (d), and soil volumetric water content at 3.8 cm depth (e) and 7.6 cm depth (f). Black symbols denote the measures performed in controls and white symbols indicate the measures performed in CCS plots. In all panels (with exception of rainfall in panel D), the circles indicate the measurements conducted in the forest site and the triangles indicate the measurements performed in the clearing site. For rainfall, the bars in solid black and white bars denote the rainfall within the forest at the different weeks of the experiment, while the dashed black and white bars are the rainfall values in the clearing.
Accumulated rainfall during the oak growing season was significantly higher in the clearing than within the forest (17 % higher) and, in both habitats, rainout shelters significantly reduced rainfall within CCS plots (Table 2). Nevertheless, differences in rainfall between CCS plots and controls were larger within the forest. In average, CCS plots in the clearing received 14-17 % less rainfall than their respective controls, while rainfall in CCS plots located beneath the forest canopy was reduced by 18-24 % (Figure 2D). These differences in rainfall concurred with the observed differences in soil volumetric water contents. The values of this latter variable were significantly higher in the clearing than in the forest but, in both habitats, soil volumetric water contents were lower in CCS plots than in controls (Table 2). At both soil depths (3.6 cm and 7.8 cm), controls and CCS plots from the clearing always had higher soil water contents than their equivalent experimental units located within the forest (Figure 2E, 2F).
Seedling emergence, survival and growth. The tetrazolium test indicated that all ungerminated acorns recovered by the end of the experiment were not viable. Thus, we assumed that germination was no longer possible after the last monitoring date. Both in the forest and the clearing, seedling emergence rates stabilized 40 days after beginning the experiment and were significantly higher in controls than in CCS plots (Figure 3A). However, no differences were found between habitats within climate treatments (Figure 3A).
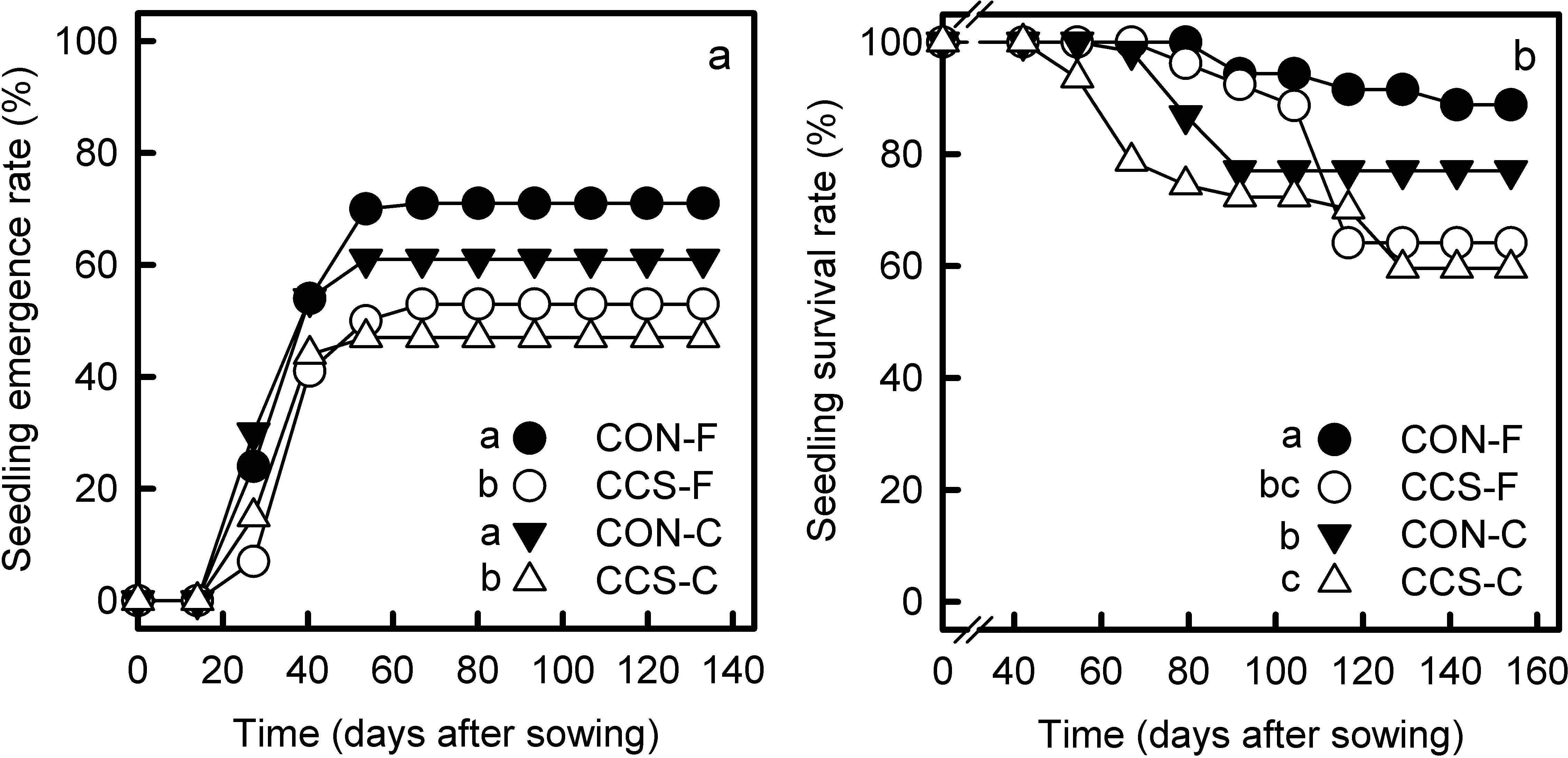
Figure 3 Emergence rates (a) and survival rates (b) of Q. eduardii seedlings in the different combinations of climate treatments and habitat types (CON-F = controls within the forest; CCS-F = CCS plots within the forest; CON-C = controls in the clearing; CCS-C = CCS plots in the clearing). Different letters on the side of treatment symbols indicate significant differences (critical α of Gehan-Wilcoxon tests for pairwise comparisons = 0.05).
Seedlings that grew in controls from the forest had the highest survival rates, as compared with seedlings from all other combinations of habitat types and climate treatments (Figure 3B). Control seedlings from the clearing had higher survival rates than those from their respective CSS plots (Figure 3B). Although survival rates in both habitats were lower in CCS plots than in controls, these differences were larger within the forest than in the clearing (Figure 3B).
Stem height of seedlings increased with time in all combinations of climate treatments and habitat types, but these relationships differed among them (F (8,2709) = 11,305.001, p < 0.001, R 2 = 0.737). Control seedlings within the forest grew significantly faster (i.e., they reached higher stem heights in fewer days; p < 0.001 for comparisons of both β 0 and β 1) than seedlings from all the other experimental treatments (Figure 4). No differences in the relationships between height and time were found among the other treatments (Figure 4).

Figure 4 Relationships between stem height and time for seedlings of Q. eduardii that survived until the end of the experiment in the different combinations of treatments and habitat types. These relationships are provided separately for the forest (a) and the clearing (b) to improve the visibility of results, but all of them come from the same multiple regression analysis. Each panel show the relationships obtained for controls (solid symbols-solid line) and CCS plots (empty symbols-dashed line). Regression functions describing each relationship are provided within the respective panels.
Functional responses of seedlings. Unfolding rates of the first leaf differed across seedlings from the different habitat types. Leaf unfolding occurred earlier in the clearing than within the forest, but no differences between climate treatments were found within each habitat (Figure 5B). Conversely, leaf senescence occurred earlier in the forest canopy than in the clearing. Senescence rates within the forest were higher in controls than in CCS plots, while no differences were found between climate treatments of the clearing (Figure 5B).

Figure 5 Unfolding (a) and senescence (b) rates of the first leaf in Q. eduardii seedlings that grew in the different combinations of climate treatments and habitat types (CON-F = controls within the forest; CCS-F = CCS plots within the forest; CON-C = controls in the clearing; CCS-C = CCS plots in the clearing). Different letters on the side of treatment symbols indicate significant differences (critical α of Gehan-Wilcoxon tests for pairwise comparisons = 0.05).
Photosynthetic photon flux densities (PPFD) measured on seedling leaves were significantly higher in the clearing than beneath the forest canopy (1,885.544 ± 4.737 vs. 872.711 ± 3.956; values are average μmol m-2 s-1 ± 95 % IC), but no differences in PPFD were found between climate treatments (Table 3). This indicates that, in both habitats, open-top chambers and rainout shelters had little effect on the light environment of CCS plots. Leaf temperatures (LT) differed between climate treatments and habitat types, and significant effects were found for the interaction of these factors (Table 3). Seedlings from the clearing reached higher LT values than those from the forest but, in both habitats, values of this variable were higher within CCS plots than in controls (Figure 6A). The effective quantum yield of photosystem II (ɸ PSII ) also differed between climate treatments and habitat types (Table 3). In both habitats, control seedlings had higher ɸ PSII than those from CCS plots (Figure 6B). However, irrespective of the climate treatment, ɸ PSII values were higher in seedlings that grew within the forest than in seedlings from the clearing (Figure 6B). Percent water contents (PWC) and specific leaf areas (SLA) of seedlings differed between climate treatments and habitat types (Table 3). In both habitats, seedlings from controls had higher PWC (Figure 6C) and SLA (Figure 6D) than seedlings from CCS plots. Nevertheless, these differences were larger within the forest than in the clearing (Figure 6C, 6D).
Table 3 Results of two-way ANOVAs used to assess the effects of climate treatments, habitat types and the interaction of these factors on variables measured on seedling leaves, including photosynthetic photon flux deity (PPFD), leaf temperature (LT), effective quantum yield of photosystem II (ɸ PSII ), percent water content of leaves (PWC) and specific leaf area (SLA). In all cases, significant effects are indicated with an asterisk (critical α = 0.05).
Response variable | Climate treatment effect | Habitat type effect | Precipitation |
---|---|---|---|
PPFD | F (1,56) = 105825.657* | F (1,56) = 1.279 | F (1,56) = 2.048 |
LT | F (1,56) = 1734.831* | F (1,56) = 706.824* | F (1,56) = 167.957* |
ɸ PSII | F (1,56) = 1199.934* | F (1,56) = 2811.157* | F (1,56) = 247.207* |
PWC | F (1,56) = 1269.959* | F (1,56) = 91.005* | F (1,56) = 438.355* |
SLA | F (1,56) = 835.792* | F (1,56) = 61.188* | F (1,56) = 18.040* |

Figure 6 Average values (± 95 % CI) of foliar temperature (a), effective quantum yield of photosystem II (b), percent water content (c) and specific leaf area (d) of Q. eduardii seedlings that grew in the different combinations of climate treatments and habitat types (types (CON-F = controls within the forest; CCS-F = CCS plots within the forest; CON-C = controls in the clearing; CCS-C = CCS plots in the clearing). Different letters above the bars indicate significant differences between experimental treatments (critical α of Tukey for pairwise comparisons = 0.05).
Discussion
Our results indicate that open-top chamber and rainout shelters properly simulated the temperature and rainfall levels expected to occur during the oak growing season in the short term (2040), also indicating that these changes can negatively affect the early life-cycle stages of Q. eduardii. Indeed, as the intensity of climate change simulations differed between the forest and the clearing, this could have caused the differential seedling responses that we observed between these habitats. These results, however, must be taken with caution because we just considered a single forest, a single clearing and a single growing season, while these effects of climate change are likely to fluctuate in space and time due to variability across sites and interannual oscillations in temperature and rainfall. Considering these caveats, in the following sections we discuss why these climatic differences between habitats occurred and their consequences for recently emerged oak seedlings.
Climate change simulation. The lower air and soil temperatures recorded within the forest, as compared with the clearing, can be attributed to tree canopies, which intercept large fractions of solar radiation and this reduces heat loading in the understory (You et al. 2013). This may also explain the lower warming reached with open-top chambers in the forest, where temperature differences between CCS plots and controls were smaller than in the clearing. Nevertheless, in both habitats, the increases in temperature simulated with open-top chambers concurred with the values predicted by climate change models for 2040. These differences in temperature between habitats and climate treatments can also explain why air relative humidity was lower in warmer experimental units, as these two climatic variables are inversely related between them (Hardwick-Jones et al. 2010).
Rainfall reduction induced with rainout shelters in the clearing concurred with the values predicted for the study site by climate change models. However, rainfall reduction in CCS plots located within the forest was more intense than predicted by these models. On this issue, it is important to note that canopies of well-preserved oak forest can retain up to 25 % of rainfall (Bahmani et al. 2012) and, in our case, it seems that this canopy effect was added to that of rainout shelters. Thus, although our experiment was not intended to provide predictions about what will occur in forest understories, these results suggest that soil beneath forest canopies will receive much less rainfall than it currently receive if climate change intensifies.
The lower soil water contents within the forest can be linked with the rainfall differences recorded between habitat types, while rainout shelters would explain why soil water contents were lower in CCS plots from both habitats. Further, as increasing soil temperature can enhance water evaporation (Parlange et al. 1998), this may have also contributed to reduce soil water content in CCS plots. Nevertheless, the lower water content in the soil of the forest site suggests that the combined effects of raising temperature and decreasing rainfall could cause more intense soil water deficits in understory habitats than in man-induced clearings.
Seedling emergence and survival. The current climatic differences between the forest and the clearing seem not affect the emergence of Q. eduardii seedlings. However, seedling emergence rates were lower in CCS plots from both habitats, suggesting that climate change will impair this process. This effect can be associated with the elevated recalcitrance of acorns, which quickly lose viability when exposed to conditions that promote water loss (Zavala-Chávez 2001). This was confirmed with the tetrazolium tests applied to ungerminated acorns. Therefore, it can be proposed that the elevated temperatures and lower soil moisture contents of CCS plots may have accelerated their desiccation and, consequently, reducing seedling emergence.
The highest survival of Q. eduardii seedlings in controls from forest suggests that, under the current climatic conditions, this tree species prefers to recruit in shaded understory habitats rather than in open areas. This concurs with the recruitment patterns of most oak species from Asia, Europe and North America (Broncano et al. 1998, Li & Ma 2003, Puerta-Piñero et al. 2007, Gómez-Aparicio et al. 2008, Badano et al. 2015). In our case, however, it is important to highlight that survival in controls from the clearing was about 80 % by the end of the growing season. This reinforces the suggestion that Q. eduardii, unlike other oaks form seasonally dry forest of Mexico, is a pioneer tree that colonize deforested sites (Alfonso-Corrado et al. 2007).
In both habitats, the seedlings of Q. eduardii had lower survival in CCS plots. These are not breaking news, as several authors have previously reported that climate change will affect tree recruitment (e.g.,Hanson & Weltzin 2000, Walck et al. 2011). However, the direction of these effects (positive or negative) and the factors behind them (changing temperature or rainfall) seem to vary across climate types. Tree recruitment in alpine and boreal ecosystems is mainly constrained by low temperatures, and some studies indicated that temperature increases of 1.8-2.0 °C may allow tree species to colonize habitats that currently are below their thermal tolerance thresholds (Batllori et al. 2009, Lindner et al. 2010). Conversely, tree recruitment in rainforest is mainly driven by water availability, and reductions of 10-20 % in annual rainfall are predicted to increase tree mortality and reduce seedling establishment (Engelbrecht et al. 2007, Malhi et al. 2009). In seasonally dry forests, climate change models predict higher temperature and lower rainfall, and our results suggest that the combined effect of these two factors can cause water deficits that will reduce the emergence and survival of tree seedlings. Indeed, as the differences in seedling survival between controls and CCS plots were larger in the forest than in the clearing, it can be proposed that the magnitude of these effects will be greater in understory habitats.
Seedling development. The fastest stem elongation of control seedlings within the forest, as compared with all other experimental treatments, indicates that shaded understory environments promote the development the early life-cycle stages of Q. eduardii under the current climatic conditions. Seedling growth in this habitat, however, was negatively affected by climate change induction. Some previous studies have indicated that water stress is the main factor constraining growth of oak seedlings, even when they are established in shaded habitats (Aranda et al. 2005, Mahall et al. 2009). Thus, the reduced growth of these seedlings could be due to the lower soil water content that resulted from higher temperatures and lower rainfall in CCS plots.
In the clearing, seedling growth in controls was much lower than that observed in controls from the forest. This concurs with the results of most studies that have compared the performance of oak seedlings between shaded and sunny habitats, which showed that growth rates are lower in open areas (Broncano et al. 1998, Puerta-Piñero et al. 2007, Quero et al. 2006, Gómez-Aparicio et al. 2008). Further, as seedling growth in the clearing was similar between controls and CCS plots, it seems that the current environmental conditions in this habitat are so hard that climate change will have negligible effects on stem elongation of Q. eduardii seedlings.
This slower stem growth of seedlings in both climate treatments from the clearing, as well as in CCS plots from the forest, may be related with the elevated energetic investments that these early life-cycle stages of oaks must perform to maintain other aboveground structures. The earlier unfolding of the first leaf in seedlings from the clearing may have been promoted by the elevated light levels recorded in this habitat (Fu et al. 2013). However, the shortest life-span of leaves (i.e., differences between unfolding and senescence rates) was recorded in control seedlings from the forests. These results suggest that Q. eduardii seedlings that grew under the current climatic conditions beneath the forest canopy would prioritize resource allocation to stem growth rather than leaf maintenance. Conversely, those seedlings that grew under harsher environmental conditions (i.e., in the clearing and CCS plots from the forest) would prioritize the maintenance of their leaves for longer periods. This concurs with the proposal that plants subjected to elevated physical stress extent the life-span of their leaves as much as possible because replacing these structures implies elevated energetic costs (Chabot & Hicks 1982).
Climate manipulation also induced several other foliar responses in Q. eduardii seedlings. Both in the forest and the clearing, seedlings from CCS plots had lower leaf percent water contents (PWC), specific leaf areas (SLA) and effective quantum yields (ɸ PSII ) than control seedlings. These functional responses suggest that the early life-cycle stages of this oak are sensitive to climate change irrespective of the habitat in which they are establishing. The lower PWC of seedlings from CCS plots may be due to their higher leaf temperatures, which could have enhanced water loss through transpiration (Quero et al. 2006). Thus, the smaller SLA of these seedlings may be a response addressed to prevent excessive water loss. This is a common response in tree seedlings growing under water deficits, which increase the thickness of the leaf cuticle and epidermis to reduce transpiration, while they reduce their leaf surface area to minimize contact with the external environment and concentrate larger fractions of photosynthetic biomass in smaller surfaces to maintain positive carbon balances (Quero et al. 2006). The smaller SLA of seedlings from CCS plots may have prevented the dropping of PWC below wilting thresholds, allowing a few of them to survive under warmer and drier conditions. Nevertheless, these seedlings had lower ɸ PSII than control seedlings, which indicates that climate change may impair this light-dependent process of photosynthesis. Several authors reported that thermal and water stress impair the capability of plants to convert light into chemical energy for fixing carbon (i.e., NADPH and ATP), which ultimately reduces their growth and survival (Xu & Zhou 2006, González-Salvatierra et al. 2013, Aragón-Gastélum et al. 2014, Badano et al. 2015). Thus, as these negative effects of warming and drought on seedlings were more pronounced in the clearing than in the forest, this may contribute to explain why their survival and growth rates were lower in the former habitat.
Conclusions
Considering that the emergence and survival of oak seedlings is currently constrained by elevated temperatures and water shortage conditions that occur during their growing season (Alfonso-Corrado et al. 2007, González-Salvatierra et al. 2013, Badano et al. 2015), our results indicate that climate change will magnify these effects and negatively affect these early recruitment processes of oaks. Our findings also indicate that oak seedlings subjected to higher temperatures and lower water availability can display functional responses that, despite reducing their growth, may confer them some tolerance to these climatic changes. Further, the larger differences in seedling survival between climate treatments that we found within the forest, as compared with those that occurred in the clearing, suggest the replacements of individuals through natural regeneration could be more strongly reduced in well-preserved understory habitats than in man-disturbed areas. However, as mentioned earlier, these results must be taken with caution because these effects can vary spatially and temporally, and extensive studies embracing longer periods and a larger number of species are required to fully determine how climate change will affect tree recruitment in seasonally dry forests.