1. Introduction
Considering renewable resources (e.g., solar and eolic, etc.), biomass is the only organic carbon raw material that could be converted to value-added products. Along past few decades, research on the production of value-added chemicals, alternative fuels and platform compounds from lignin has grown rapidly attesting its importance in biorefineries schemes. Lignin accounts for 10-35% by weight, up to 40% by energy in biomass. Then, chemical and fuels production from lignin could significantly improve the economics of biorefinery process (Li et al., 2015). Hydrodeoxygenation (HDO) is the most efficient method for bio-oil upgrading as it allows to decrease original oxygen content (typically 10-40 wt%) to essentially total elimination under operating conditions similar to those used in commercial hydrotreaters for the production of oil-derived diesel. High oxygen content in raw biomass derived-oils imparts undesirable physical and chemical properties as high viscosity, corrosiveness and thermal instability among others, precluding their direct use (Bui et al., 2011a). Guaiacol (GUA) well represents aromatic oxygenated species present in biomass pyrolysis oil (Qi et al., 2007) or lignin depolymerization products (Chio et al., 2019). Several investigations on GUA and other oxygenates HDO have been addressed by using alumina-supported CoMo (Bui et al., 2011b; Bui et al., 2009; Tavizón-Pozos et al., 2019) or NiMo (Kaluža et al., 2019; Lin et al., 2011) sulfided formulations finding interesting results. Thus, obtaining CoMo/Al2O3 solids of enhanced catalytic properties (activity and selectivity, stability under reaction conditions, etc.) is always demanded.
The use of organic additives during hydrodesulfurization (HDS) catalysts preparation has resulted in enhanced organo-S removal activity during oil-derived streams processing. It is well-accepted that complexing organic compounds could enhance Mo-promoter (Ni or/and Co) interaction during catalyst sulfiding that resulting in materials of increased HDS activity (Lélias et al., 2009; van Haandel et al., 2017). Chelation allows promoter sulfidation to temperatures high enough to permit efficient integration of sulfidic Co or/and Ni species to then already formed MoS2 (or WS2), thus optimizing promoted phases (“NiMo(W)S” or “CoMo(W)S”) formation. On the other hand, regarding non-complexing species as HDS catalysts additive no general consensus on the origin of their beneficial influence has been reached so far. It appears that enhanced HDS activity could be originated by a number of factors (Escobar et al., 2009; Escobar et al., 2018; Nicosia & Prins, 2005a; Nicosia & Prins, 2005b). For instance, for glycols-modified P-doped CoMo/Al2O3 formulations it has been proposed that interaction of organic additive with both basic OH groups and coordinatively unsaturated Al3+ sites on alumina surface could partially prevent Co diphosphopentamolybdate Strandberg heteropolyanions (Catita et al., 2017) decomposition during their deposition on the carrier (Nicosia & Prins, 2005a). Thus, close proximity of Co and Mo in those complexes could result in increased amount of highly active mixed “CoMoS phase” during sulfiding. Beneficial influence of ethylene glycol when simultaneously impregnated with Ni, Mo and P over alumina support has been documented (Escobar et al., 2009) as well. Again, enhanced formation of well-promoted “NiMoS” phase due to decreased support-impregnated Mo interaction was invoked to explain enhanced activity in both dibenzothiophene (DBT) and straight-run gas oil HDS. Recently, we reported (Escobar et al., 2018) on using saccharose as precursor of Mo6+ species reducing agents (monosaccharides) rendering Mo-blue species that after sulfiding resulted in alumina-supported P-doped NiMo catalyst of improved HDS. Under synthesis conditions and by reducing Mo6+ species monosaccharides could be converted to complexing organic acids that could possibly interact with Ni promoter being also able to decrease deposited species-carrier interaction by carbonaceous deposits formation. Then, it seems that positive influence of non-complexing organic additives on the S-removal activity of corresponding catalysts could be related to several independent phenomena.
Comparative studies (Vatutina et al., 2020) using CoMo formulations over multi-walled carbon nanotubes (MWCNT) found that chelating citric acid was more efficient than diethylene glycol addition in improving DBT HDS and quinoline hydrodenitrogenation. Decreased support-Mo interaction provoked by the later additive was not very effective in promoting activity as impregnated phases were not strongly bonded to MWCNT. On the other hand, triethylene glycol and thioglycolic acid addition resulted in decreased active phase aggregation (diminished MoS2 slab length and stacking in CoMoP/Al2O3 catalysts) that was reflected in augmented activity in both toluene hydrogenation and straight-run gas oil-light cycle oil mixture HDS (Humbert et al., 2021). Clearly, the effect of various organic additives, either chelating or not, on HDS catalysts performance depends upon specific interactions with the deposited phases (Mo, W, etc.) and promoters (Ni, Co, etc.) under distinctive preparation conditions (concentration, pH, type of support, etc.). MoS2 particles morphology, as determined by organics addition, could also strongly influence selectivity by exposing preferential edges. For example, sulfur vacancies on S-edge of CoMoS2 catalysts had higher selectivity for heteroatoms removal over that to hydrogenation (Zheng et al., 2021).
During the present investigation Al2O3-supported P-doped CoMo catalysts were prepared by adding complexing and non-chelating organic modifiers (CA and SA, respectively) at two different preparation stages. Oxidic materials were studied by using several textural, structural and surface characterization techniques. Also, corresponding sulfided catalysts were studied by HR-TEM to determine their morphology (MoS2 slabs length and stacking).
2. Materials and methods
2.1. Catalysts synthesis
Al2O3 powder was obtained by annealing (500 ºC, 5 h under static air) commercial Pural SB sol-gel pseudo-boehmite (from Sasol, obtained from Al-alkoxides). Oxidic catalyst precursors were prepared by one-pot impregnation of Mo, Co and phosphorous (at 12, 3 and 1.6 wt%, respectively) by pore-filling through an aqueous solution where MoO3 was digested (at ~80 ºC) in diluted H3PO4. H2O in excess (typically 250 ml of starting solution to impregnate 5 g of alumina carrier) was used to accelerate dissolution and digestion of molybdenum salt. After ~4 h cobalt acetate (C4H6CoO4•4H2O) was added, the solution being then digested by additional 4 h (~80 °C). The described one-pot impregnation method was chosen as it constitutes a readily scalable methodology used during commercial catalysts preparation. It includes cheap industrial precursors easily affordable for industrial catalysts manufacturing. To determine the influence of organic modifiers (saccharose, SA, at SA/Co=1 (Escobar et al., 2018) or citric acid, CA, at CA/Co=2 mol ratio (Escobar et al., 2008) added at different preparation steps two methodologies were followed. In method I SA or CA were directly added to the already prepared Co-Mo-P aqueous solution (at ~80 ºC, pH~2) to simultaneously (one-pot) impregnate the corresponding amount of Al2O3 carrier. Immediate color change from wine-color to almost black (signaling complexes formation) was observed for the SA-modified impregnating solution. Impregnated materials were allowed to stand overnight at room temperature in a capped beaker to permit diffusion of deposited species into the support porous network. Materials were dried at 120 ºC (2 h), samples SAI and CAI, respectively. Regarding the second preparation methodology, the carrier was firstly impregnated by pore-filling with aqueous SA or CA solutions at concentration again corresponding to SA/Co= 1 and CA/Co=2 mol ratio, solids being then dried at aforementioned conditions. Subsequently, Co-Mo-P phases were simultaneously deposited over the modified supports by pore-filling impregnation. Resulting materials were then dried at 120 ºC (2 h), samples SAII and CAII. To preserve organic additive integrity high-temperature annealing was avoided in all cases. A reference alumina-supported Co-Mo-P impregnated reference formulation was also prepared. This solid was dried (120 ºC, 2 h, sample CMd), a portion being calcined at 400 ºC (4 h, solid CMc). Supported sulfidic catalysts were obtained by submitting approximately 0.3 g of ground (U.S. mesh size 80-100, ~165 μm average particle diameter) impregnated precursors to treatment at 400 °C (heating rate 6 °C min-1) under H2/H2S (Praxair) at 50 ml min-1/6 ml min-1 ratio during 2 h.
2.2. Materials characterization
Textural properties of support and Co-Mo-P impregnated solids were determined by N2 physisorption (at -196 ºC) in a Micromeritics Tristar II 3020 apparatus after ultra-high vacuum degassing at ~300 ºC) for 2 h. Surface area and pore size distribution of studied materials were determined by BET and BJH (adsorption branch data) methods, respectively. Thermogravimetric analysis (TGA) of impregnated samples were carried out through a TA instruments Q2000 equipment. 15-50 mg samples were analyzed in the 30-1000 ºC temperature range (heating ramp of 0.166 ºC s−1). Differential thermogravimetric (DTG) profiles were obtained through derivatives of corresponding TG curves. Also, differential scanning calorimetry (DSC) profiles of various samples prepared with organic additive were obtained through a Q2000 equipment from TA instruments.
UV-vis diffuse reflectance spectroscopy (DRS) analyses of impregnated samples were carried out in a Perkin Elmer Lambda 35 spectrophotometer equipped with integration sphere. Spectra were acquired in the wavelength range from 200 to 1100 nm. Spectralon signal was subtracted as baseline. Supported Co-Mo samples (~100 mg put in a quartz cell) were studied by temperature programmed reduction (TPR, in the 25-850 ºC range, 10 ºC/min heating rate) using an ICID SRyC-2 apparatus equipped with a thermal conductivity detector. To that end, a H2/N2 mixture (at 1/9 L/L, 20 cm3/min total flow) was used. X-ray photoelectron spectroscopy (XPS) analysis was carried out using a K-alpha Thermo Fischer Scientific spectrometer with a monochromatic Al Kα radiation (1486.6 eV) as X-ray source that was micro-focused at the source to give a 400 microns diameter spot size on the sample. XPS high-resolution spectra were collected using analyzer pass energies of 20 eV. To compensate effects related with charge shift, C1s peak at 284.6 eV was used as internal standard.
Sulfided catalyst (see 2.1. Catalysts synthesis section) were characterized by high-resolution transmission electron microscopy (HRTEM) performed in a Titan 80-300 microscope with a Schottky-type field emission gun operating at 300 kV. The point resolution and the information limit were better than 0.085 nm. HRTEM digital images were obtained using a CCD camera and Digital Micrograph Software from GATAN. In order to prepare the materials for observation, the powder samples were ultrasonically dispersed in ethanol and supported on lacey carbon coated copper grids. GATANTM software was used during determination of MoS2 particles morphology (slab length and stacking degree). Average length and stacking number of MoS2 slabs (Lavg. and Navg., respectively) were statistically estimated through measurements carried out in ~300 MoS2 slabs per sample through the following equations:
where ni is the number of slabs in a given range of length or stacking (Li and Ni, respectively) and t is the total number of analyzed MoS2 slabs. MoS2 phase aspect ratio, ArMoS2 (1/nm), was defined as:
MoS2 edge-to-corner ratio (fe/fc)MoS2 was calculated through the following equation:
where Moe and Moc stands for Mo4+ atoms in edges and corners of hexagonal MoS2 particles, respectively.
Dispersion of molybdenum atoms in MoS2 crystals was determined from corresponding hexagonal geometrical model (Kasztelan et al., 1984). Thus:
where DMoS2 is MoS2 dispersion, Moe and Moc as previously defined meanwhile Mot stands for total molybdenum atoms (as determined by slabs size); ni is the number of atoms along one side of a molybdenum sulfide slab as determined from its length, L=0.316×(2ni-1), in nm, and t is the total number of slabs analyzed from HR-TEM corresponding micrographs during the statistical counting. Interesting correlations between MoS2 particles morphology (slab length and stacking) and their activity and selectivity in HDO reactions have been reported in the past (Liu et al., 2018; Wu et al., 2016; Wu et al., 2019 Yang et al., 2008).
3. Results and discussion
3.1. Materials textural characterization
Textural analyses of samples modified by organic additives were not carried out as presence of those agents in non-calcined solids precluded efficient parameters determination. N2 physisorption isotherms (at 196 ºC) of alumina support and corresponding impregnated materials dried and calcined (CMd and CMc, respectively) belonged to type IV, according to IUPAC classification (Figure S1) (Leofanti et al., 1998). Adsorbed N2 volume clearly decreased after impregnated phase deposition that effect being more evident for the dried sample where remains from impregnation step could exist on the carrier surface. All studied solids had type H1 hysteresis, characteristic of materials consisting of particles crossed by nearly cylindrical channels or made by aggregation (consolidated) or agglomeration (unconsolidated) of spheroidal particles. Surface area (SgBET), pore volume (Vp), and average pore diameter (Dp, assuming cylindrical pores) of solids studied are shown in Table S1. Loss of SgBET of CMc sample (respecting that of bare Al2O3 support) was higher than expected by assuming homogeneous deposition of oxidic non-porous impregnated phases (around 0.267 g g-1 of support) where a final surface area of ~183 m2 g-1 could be expected (instead of 149 m2 g-1 actually determined). That suggested some partial plugging of the carrier smaller pores where ~19% of pristine support surface area was lost after oxidic Co-Mo-P phases deposition. Expectably, that loss was much higher for the dried impregnated solid CMd (31%). To determine which pore size distribution profile (PSD, from BJH methodology) obtained by using either adsorption or desorption data branch better fit the actual ones, corresponding cumulative surface area values were compared to those obtained through the BET method. Values from adsorption branch data, SgBJHA, were closest to SgBET ones (Table S1). Thus, PSD profiles from those data were deemed the most suitable in describing the actual porous network, Figure S2. Pores of diameter <4.5 nm present in the pristine alumina carrier were almost totally absent in CMc profile after Co-Mo-P phases deposition in full agreement with the surface area loss as to that of the Al2O3 support (Table S1). For impregnated materials shift of hysteresis closing to higher relative pressure (see Figure S1) was in full agreement with smaller pores elimination. CMc PSD profile shifted to ~9.4 nm as to that of corresponding support (maximum at ~8.4 nm). Pore dimensions of our impregnated materials seemed to be adequate for their potential application as catalysts for guaiacol (kinetic diameter ~0.67 nm Lee at al. (2016) hydrodeoxygenation where effective reactants/products pore diffusivity strongly depends on pore size, porosity and tortuosity of catalytic particles porous network.

Figure 1 Differential thermogravimetric analysis profiles of various alumina-supported Co-Mo-P impregnated materials preparedwith organic additive. (a): CAI; (b): CAII; (c): SAI; (d): SAII.
Table 1 Absorptions maxima (nm) from UV-vis DRS (Schuster-Kubelká-Munk function) spectra of alumina-supported P-doped CoMo oxidic materials prepared with and without organic additives (Figure 3).
Sample | Mo6+(Th) | Mo6+(Oh) | Co2+(Th) | Co2+(Oh) | Mo-blue |
---|---|---|---|---|---|
CMd | 250 | 354, 396 | 560 | 521 | |
CMc | 254 | 335 | 540, 595, 645 |
||
CAI | 250 | 312 | 519, 785 | ||
CAII | 240 | 275 | 524, 722 | ||
SAI | 253 | 354 | 521 | 401, 692, 868 |
|
SAII | 271 | 347 | 518 | 400, 700, 867 |
3.2. Thermal analysis (TG-DTG-DSC) of impregnated solids
For all studied materials weight losses up to 100 ºC were attributable to water evaporation, Figure S3. The main losses for solids modified by organic additives after H2O elimination are shown in Table S2. Temperature ranges for various weight losses were based on corresponding DTG profiles (Figure 1). CAI and CAII had decomposition/combustion steps at similar temperature intervals (Table S2) in spite of higher weight losses observed for the latter. This trend was alike to that previously registered for Al2O3-supported NiMo formulations were enhanced losses were observed for the sample where citric acid was impregnated over the pristine carrier prior to Ni-Mo-P phases deposition (Escobar et al., 2017) suggesting higher carbonaceous remains-support interaction in the CAII formulation.

Figure 3 UV-vis DRS (Schuster-Kubelká-Munk function) spectra of alumina-supported P-doped CoMo oxidic materials prepared with and without organic additives. (a): CMd; (b): CMc; (c): CAI; (d): CAII; (e): SAI; (f): SAII.
Table 2 Maxima of signals from temperature-programmed reduction profiles of P-modified CoMo/Al2O3 materials prepared (Figure 4).
Sample | Mo6+(Oh) to Mo4+ (ºC) |
Co2+(Oh) to Co0 (ºC) |
Mo6+(Oh) in Al2(MoO4)3 to Mo0 (ºC) |
Mo6+(Th) to Mo0 (ºC) |
---|---|---|---|---|
CoMod | 521 | - | 708a | 775c |
CoMoc | 502 | - | 726a | 770c |
CAI | 504 | 648a | 727b | 772c |
CAII | 504 | 645a | 733b | 772c |
SAI | 520 | 615b | 750a | - |
SAII | 500 | 639b | 745a | - |
a: hump; b: shoulder; c: and beyond
Under the highly acidic (pH~2) conditions of solutions used during CAI impregnation weakly acidic CA (pKa values of 3.1, 4.7, and 6.4, for first, second and third ionization, respectively) could hardly be deprotonated that precluding interaction of acetate moieties with coordinatively unsaturated sites of surface Al3+cations. Losses between 100 and 280 ºC could include contributions from support dehydroxylation (surface and structural OH groups (Escobar et al., 2000) elimination of water coordinatively bonded to cobalt acetate (Grimes & Fitch, 1991) and acetates decomposition as well. Combustion of organic remains strongly bonded to the carrier could provoke the losses observed at higher temperature. Onset of signal at 780 ºC for CAII absent in CAI (DTG profiles, Figure 1), could be attributable to MoO3 melting/sublimation (Braun et al., 2005) pointing out to Mo6+ species of decreased interaction with the support as to those over CAI. Although SAII DTG profile (Figure 1) had features rather similar as to those of samples prepared with CA, SAI had distinctive features where a low temperature signal at around 205 ºC was identified. Saccharose could be rapidly hydrolyzed under pH conditions of Co-Mo-P impregnating solution (SAI sample) forming glucose and fructose. Both of these monosaccharides could polymerize at the mentioned temperature (Őrsi, 1973) producing oligo-and poly-saccharides that could be further decomposed by combustion at more severe conditions. Organics condensation and decomposition reactions could be accelerated by extant deposited Mo and/or Co species (Braun et al., 2005; Eggleston et al., 1996) those then taking place at lower temperature as to that observed for pure monosaccharides (Őrsi, 1973). SA hydrolysis could not occur when dissolved in sole water being then the pristine disaccharide directly impregnated on the alumina carrier prior to Co-Mo-P phases deposition (solid SAII). Interestingly, in SAI case onset of the weight loss related to MoO3 sublimation started at lower temperature (675 ºC) as to that observed for the rest of studied solids suggesting weakened Mo species-support interaction. Excepting absence of signal at ~380 ºC, SAII DTG profile was very similar to those of CA samples.
Regarding DSC analysis, endothermal signal around 95-110 ºC in all samples impregnated with organic additives was related to water evaporation (Stevulova et al., 2017), Figure 2. Higher intensity of that peak in CA-modified samples was in agreement with their enhanced H2O content, as to those of solids prepared with SA (see corresponding TG analysis in Figure S2). Endothermal humps at ~165 ºC in SA-containing samples (Figure 2 (a)) could be related to the disaccharide melting (Beckett et al., 2006). Exothermal signals at ~235 ºC in SA-containing samples (more notable in SAII) suggested organic remains in weaker interaction with the supports as to those on CA-modified materials where that combustion inflexion was registered at higher temperature (see CAI profile, for instance), in agreement with that observed by DTG (Figure 1). Thermal decomposition of organic additives under air resulted in hydroxyls oxidation and consequently led to enhanced number of carbonyl groups and carboxylate formation (Fagerson, 1969; Stevulova et al., 2017).
Finally, exothermal signals at around ~440 ºC in all samples studied fully agreed with decomposition of more stable carbonaceous deposits as also registered by corresponding DTG analyses (humps, Figure 1). In this line, decompositions at ~438 ºC have been attributed to oxidation of charred residues (Stevulova et al., 2017).
3.3. UV-vis DRS spectroscopy studies
Maxima of main absorptions from UV-vis spectra of various prepared materials (Figure 3) are shown in Table 1.
Tetrahedral molybdenum (Mo6+(Th)) appeared at rather similar position for all materials (240-254 nm, column 2, Table 1) (Escobar et al., 2017) but in SAII (271 nm) suggesting then species of lower interaction with the support. Mo6+(Th) entities characterize supported stable phases refractory to sulfiding (Blanchard et al., 1998). Although Strandberg heteropolyanions (Hx P2Mo5O23(6-x)- ) (Catita et al., 2017) could exist in several protonation states over a wide pH range in the one-pot simultaneous impregnation solutions used they could decompose into PO43- and molybdates due to their strong interaction with both basic OH´s and Al3+ Lewis acid sites on Al2O3 surface (Kraus & Prins, 1996). However, organic moieties adsorption on the latter sites could passivate them diminishing molybdate-carrier interaction that being reflected in decreased tetrahedral Mo species. In the same line, by studying alumina-supported CoMo formulations it has been suggested (Nicosia & Prins, 2005a) that in materials prepared in the absence of glycols Mo6+ was mainly in tetrahedral geometry.
Much higher variations in position (radiation energy) were observed for octahedral molybdenum Mo6+(Oh) over various studied materials (column 3, Table 1). Even more, Mo6+(Oh) of different dispersion were suggested in CMd (maxima at 354 and 396 nm) as blue-shifted low-energy absorption edge has been related to smaller MoxOy domains (Weber, 1995). Annealing could favor Mo species dispersion (Stampfl et al., 1987) but, when at severe conditions, formation of Mo6+(Th) strongly interacting with the alumina carrier could also take place. Similarly, to that found when studying alumina-supported NiMo formulations modified by CA at different preparation stages (Escobar et al., 2017), CAI and CAII had high Mo dispersion as inferred from the hypsochromic shift of low energy absorption edge as to those of the rest of solids (Figure 3). Proportion of octahedral species was enhanced in SA-modified samples and CMd as well pointing out to decreased amount of tetrahedral ones due to diminished Mo-support interactions (Escobar et al., 2016). Regarding Co2+, triplet with maxima at 540, 595 and 645 nm in CMc characterized tetrahedral Co2+(Th) (4A2(F) → 4T1(P) transitions) (Ramírez et al., 2000) probably in CoAl2O4-like species occupying tetrahedral vacancies of alumina structure (Rinaldi et al., 2009). Conversely, absorption at 560 nm in CMd corresponded to octahedral Co2+ ions (Co2+(Oh)) like in inner sphere surface Co2+ complexes (Rinaldi et al., 2009). Also, absorptions in the 518-524 nm range in samples prepared with organic modifiers (peaks in CAI and CAII, shoulders in SAI and SAII) could probably be related to 4T1g(F) → 4T1g(P) electronic transition of Co2+(Oh) (Xie et al., 2007) pointing out to exchange of water ligands (in Co(H2O)6)2+ with support hydroxyl groups signaling then surface Co-Al2O3 species formation. Thus, it appeared that surface passivation by carbonaceous deposits from impregnated organics impeded formation of significant amounts of Co2+(Th) in strong interaction with the alumina carrier. Absorptions beyond 700 nm in CAI and CAII could be due to p(O2-) → eg(Co3+) electronic transitions (Dhas et al., 2015).
Visible region signals around 400 nm in SA-containing formulations could be attributed to ligand to metal charge transfer (LMCT) complexes saccharide-molybdenum where Mo atoms could be partially reduced (Escobar et al., 2018). Similar absorptions due to LMCT complexes of thioglycolic acid-Mo have been previously identified by others (Toledo-Antonio et al., 2017; Palcheva et al., 2016). Position of bands due to those LMCT complexes depends upon Mo6+ and Mo5+ cations local symmetry related to their coordination and aggregation state (Zhong et al., 2018). Higher intensity of absorption at ~400 nm in SAI as to that in SAII spectrum suggested enhanced complex formation in the former. Signals at ~700 and ~867 nm related to Mo-blue species (Shukor et al., 2010) containing partially reduced molybdenum species (Escobar et al., 2018) were clearly identified in SA-modified samples. The former band (d-d transition) indicated partial Mo6+ reduction whereas the latter was originated by intervalent charge transfer Mo5+ to Mo6+ (Gavrilova et al., 2020). It has been mentioned that position of absorption maxima in the visible region could be related to shape of Mo-blue entities. Thus, peaks at around 750 nm could be due to ring-shaped toroidal species (Figure 3) whereas those at ~450 nm could be characteristic of spherical ones (Müller et al., 1999). Then, in our SA-modified samples the latter species could be predominant. Strongly increased background absorption in visible range for SA-containing solids could be provoked by their dark coloring.
3.4. Temperature-programmed reduction of prepared formulations
Maxima of signals from TPR profiles (Figure 4) are summarized in Table 2. Low-temperature humps between 220-380 ºC for CA-modified samples suggested evolution of products from decomposition of loosely bound organic additive. The corresponding broad signals in that temperature range could be provoked by both alteration of the thermal conductivity detector by evolved species and some H2 consumption as well (González-Cortés et al., 2015; Villareal et al., 2015).

Figure 4 Temperature-programmed reduction profiles of alumina-supported P-doped CoMo oxidic impregnated materials prepared with and without organic additives. (a): CMd; (b): CMc; (c): CAI; (d): CAII; (e): SAI; (f): SAII.
Signals around 500 ºC in various profiles were attributed to reduction of Mo6+(Oh) in oxomolybdates to Mo4+, as previously reported in the case of γ-alumina-supported CoMo formulations of similar loading (Zhang et al., 2019). With the sole exception of SAI, the influence of organics addition on that signal position was no significant. The shift to higher temperature (520 ºC) observed for that sample prepared by simultaneous SA + one-pot Co-Mo-P impregnating solution deposition agreed with increased LMCT complexes formation (Escobar et al., 2010), in agreement with that found by UV-vis spectroscopy (see section 3.3.). However, increased Mo6+(Oh) dispersion in CA-modified samples (as suggested by blue-shifted absorption edge in UV-vis spectra, Figure 3) was not reflected in increased reduction temperature probably due to decreased Mo-alumina interaction provoked by support-like carbonaceous remains acting as intermediate carrier as proposed by others (Ge et al., 2014) from studies on ethylenediamine-modified CoMo/Al2O3 catalysts.
Humps in the 708-750 ºC range for studied samples could be related to reduction of Mo6+ in Al2(MoO4)3 (Qu et al., 2003). Although those molybdates are favored by high-temperature annealing (migration to octahedral vacancies in the alumina structure) it seemed that impregnating by acidic Co-Mo-P solutions (pH~2) could partially dissolve the Al2O3 network that disruption provoking then aluminum molybdate formation in non-calcined samples.
Small H2 consumption signals at 615-648 ºC in solids containing organic additive could be provoked by reduction of Co2+ in tetrahedral vacancies of γ-Al2O3 phase to Co0 (Madduluri et al., 2019; Vosoughi et al., 2017). Shift of that peak to lower temperature in SAI (615 ºC) suggested lessened Co-carrier interaction. On the other hand, absence of those consumptions in CoMod and CoMoc TPR profiles could be due to hardly reducible Co2+(Th) in cobalt aluminate domains, in full agreement with that found by UV-vis DRS (see Figure 3 and Table 1).
Signals at temperature beyond 770 ºC in all samples (but in SA-modified ones) could include contributions from Mo4+ transformation to corresponding metallic phase and Mo6+(Th) reduction of strongly interacting with the carrier (Escobar et al., 2017) as well. The most intense of those signals were observed for CAI and CMc. For the latter, high temperature annealing (400 ºC) at which the sample was submitted could favor stronger Mo-support interaction. In full agreement with that, CMd (no submitted to calcining) had much lower H2 consumption signal related to those refractory species reductions that could however exist as consequence of using acidic impregnating solutions, as previously mentioned. In the same line, when citric acid was directly added in the one-pot Co-Mo-P solution impregnating pH could slightly decrease leading to enhanced carrier dissolution. (Yin et al., 2011). Thus, proportion of refractory molybdenum could be augmented (see corresponding CAI profile in Figure 4). Absence of those Mo species in SA-modified solids points out to strong interaction of support with carbonaceous deposits that effectively passivated the alumina surface (Escobar et al., 2016). As the amount of C deposited on CA- and SA-modified solids was very similar (~0.072 g/gcat) enhanced proportion of more stable graphitic support-like carbon (Escobar et al., 2018) in the latter formulations was strongly suggested.
3.5. Mo 3d XPS studies of oxidic impregnated formulations
Mo 3d spectra of various oxidic impregnated formulations dried at 120 ºC (CMc being sole exception as it was calcined at 400 ºC) are shown in Figure 5.

Figure 5 XPS Mo3d spectra of alumina-supported P-doped CoMo oxidic impregnated materials prepared with and without organic additives.
The Mo 3d spectrum of samples prepared with no organic additive (both dried and calcined, CMd and CMc, respectively) presented Mo 3d5/2 and Mo 3d3/2 doublet with binding energy of 232.57 and 235.64 eV, respectively, attributed to Mo6+ in oxomolybdates (Pimerzin et al., 2017). Wider full width at half maximum (FWHM) of Mo3d5/2 signal of SA- and CA-containing samples as to those of non-modified solids (CMd and CMc) (Figure 5) suggested existence of more than one type of Mo species. All materials prepared with organic additive showed maxima shifted to lower binding energy (Mo 3d5/2 and Mo 3d3/2 doublet at 232.07 and 235.03 eV, respectively) pointing out to contributions from partially reduced Mo5+ species. In full agreement, others (Koyun et al., 2017) identified Mo-blue XPS signals synthesized in materials prepared in one-step solid state technique using voltammetric method. Mo 3d5/2 and Mo 3d3/2 peaks (from Mo6+) at 232.98 and 236.08 eV, respectively, accompanied by contributions from Mo5+ at lower binding energy. Also, XPS signals related to partially reduced Mo5+ (additionally to those from Mo6+) have been identified (Chong et al., 2018) in [MoO3(3,8-phenanthroline)0.5] containing Mo-blue species where Mo 3d5/2 and Mo 3d3/2 peaks were observed at about 231.3 and 234.5 eV. Interestingly, presence of Mo5+ surface species was related to dark coloring of samples, in full agreement with strongly increased background absorption in visible range for SA-containing samples (Figure 3).
In our case, shift to lower energy (Mo6+ to Mo5+) was less pronounced in CAI where at the acidic conditions of impregnating solution (pH~2) citric acid could not be deprotonated in significant proportion (Escobar et al., 2017). Thus, interaction between Mo6+ species and organics could not be very strong as the organic acid could be largely unaltered. Partial molybdenum reduction (Mo5+ species) by either citric or malonic acid addition has been reported by others (Valencia et al., 2019) in the case of SiO2-supported molybdates obtained from ammonium heptamolybdate annealing. Presence of those Mo5+ (even some Mo4+) species was attributed to organic acids oxidation by supported Mo6+. In this case, however, no direct relationship between amount of partially reduced Mo species and activity in the palmitic acid HDO was found.
It is worth mentioning that interaction between molybdenum and citric acid could take place just after Co2+ complexes formation due to higher stability of the latter species at the concentrations (~2.5 and ~1 mol l-1 of Mo and Co, respectively) and pH conditions of impregnating solutions (Escobar et al., 2008; Sun et al., 2003). In prepared solutions used for Co-Mo-P one-pot simultaneous impregnation (as in CAI case, see section 2.1. Catalysts synthesis) cobalt cations could be preferably complexated over molybdenum ones. Thus, Mo entities reduction by CA could not be extensive in agreement with diminished Mo5+ species formation found by XPS (Figure 5). In full agreement, Mo complexation by CA was enhanced when the alumina bare support was first impregnated with citric acid in aqueous solution prior to Co-Mo-P phases deposition (CAII) where the organic modifier could possibly occupy coordinatively unsaturated sites (CUS, Al3+) on Al2O3 carrier where several chelated Mo species could be identified (Escobar et al., 2017; Van Veen et al., 1989).
Regarding non chelating agents, Mo5+ species on dried alumina supported NiMo materials modified by ethylene glycol addition has been found (by XPS) (Nuzhdin et al., 2018), although no explanation for their existence was advanced in this case.
Respecting saccharide-modified solids our group has recently investigated on the effect of monosaccharides (from saccharose acid hydrolysis) as Mo+6 reductants which addition resulted in Mo-blue species formation (Escobar et al., 2018). Mo6+ (from P2Mo5O236- Strandberg heteropolyanions) reduction due to ligand to metal charge transfer (LMCT) complexes resulted in Mo-blue species that constituted suitable precursors of highly active alumina-supported sulfided NiMo HDS catalysts. Very interestingly, Mo5+ species from reduction of Mo6+ by using citric acid addition has been investigated on polymolybdates films (Jing et al., 2005) where materials activated by UV irradiation (during 30 s) had signals related to electronic transitions forming strong symmetric band in the 500-900 nm region (maximum at λ=670 nm) induced by intervalence charge transfer (IVCT, Mo5+↔Mo6+) and d-d transitions of reduced polymolybdate species (Mo-blue) which presence was confirmed by XPS. In that study. absorption at 738 nm in visible regium spectrum was assigned to [Mo14O46]10- species. In the same line, humps at 782 and 900 nm and at 720 and 865 nm in visible spectra of CAI and CAII, respectively (Figure 3) suggested extant partially reduced molybdenum species as well, as corroborated by corresponding XPS analyses (Figure 5). According to several reports, partially reduced Mo5+ species could play significant roles in HDO activity of corresponding Mo-based supported catalysts. For instance, improved catalytic activity (turn over frequency and stability) in the anisole HDO was related to active Mo oxide defects (e.g., Mo5+ species originated by post-synthesis treatment under H2/CH4 of ZrO2-supported oxidic Mo precursors) (Ranga et al., 2018). However, higher molybdenum reduction (to Mo4+) resulted less favorable (Prasomsri et al., 2014). Mo5+ species generated by MoO3 conversion either by carburization to MoOxCyHz or by reduction to MoO3-x in presence of H2 could be stabilized by lattice carbon preventing further reduction to Mo4+ by forming an oxycarbohydride phase (Prasomsri et al., 2014). Mo4+-containing species as MoO2 are completely inactive in phenolic species HDO (Prasomsri et al., 2014). On the other hand, Mo5+ species could presumably behave as Lewis acid sites (oxygen vacancies) that could weaken C-O bonds in molecules adsorbed on those active sites.
High activity and on-stream stability in the catalytic fast pyrolysis of lignocellulosic biomass was also observed in ZrO2- and TiO2-supported Mo (10 wt%) which contained over 50 wt% of partially reduced Mo species (Mo5+ and even Mo3+ in the material with titania as carrier) (Murugappan et al., 2016).
3.6. Sulfided catalysts characterization by high-resolution electron microscopy (HR-TEM)
From corresponding electron micrographs (Figure 6(a) - (f)) some changes in MoS2 particles morphology (slab length and stacking) of prepared sulfided catalysts by organics addition at different preparation stages were detected.
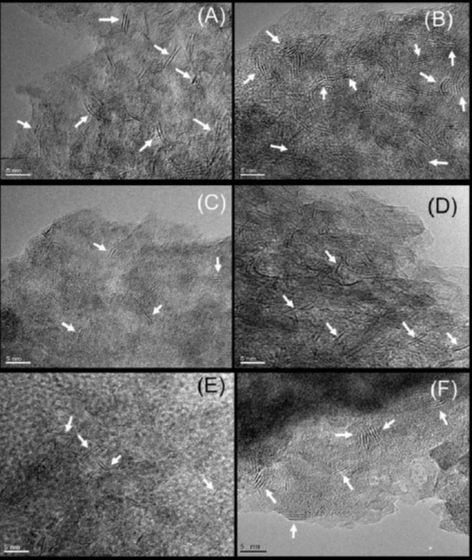
Figure 6 HR-TEM micrographs of various sulfided catalysts prepared with and without organic additives. White arrows: MoS2 particles. (a) CMd; (b) CMc; (c): CAI; (d): CAII;(e): SAI; (f): SAII.
MoS2 slabs average length of around 0.62 nm interplanar d-spacing (S-Mo-S layers in (002) plane) decreased, as to that in solids prepared with no organics, in materials modified by either CA or SA addition (Figure 7(a) and (b) and Table 3).
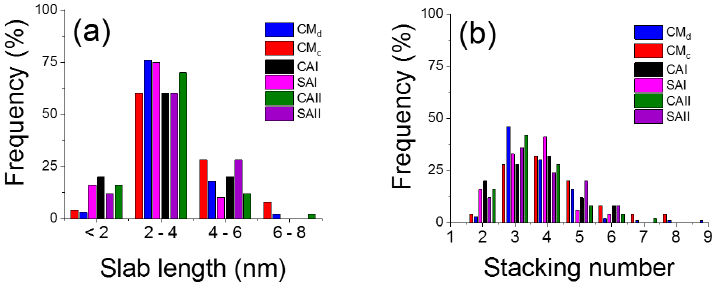
Figure 7 Statistical distribution of (a) length and (b) stacking of MoS2 slabs over various sulfided catalysts prepared, as determined from corresponding HR-TEM micrographs.
Table 3 Morphological properties of MoS2 particles on various sulfided catalysts prepared (from statistical analyses shown in Figure 7(a) and (b)).
Catalyst | aLavg.(nm) | bDMoS2 | cNavg. | dArMoS2(1/nm) | e(fe/fc)MoS2 |
---|---|---|---|---|---|
CMd | 3.84 | 0.30 | 4.3 | 1.12 | 4.50 |
CMc | 3.46 | 0.33 | 3.7 | 1.07 | 3.91 |
CAI | 3.20 | 0.36 | 3.6 | 1.12 | 3.50 |
SAI | 3.07 | 0.37 | 3.5 | 1.14 | 3.30 |
CAII | 3.16 | 0.36 | 3.5 | 1.11 | 3.44 |
SAII | 3.44 | 0.34 | 3.8 | 1.10 | 3.88 |
a Average slab length; b as estimated by Kasztelan model (Kasztelan et al., 1984) equation (5); c Average stacking; d Aspect ratio (from equation (3)); e Mo (in MoS2 slabs) edge-to-corner ratio from HR-TEM (equation (4))
Also, with the sole exception of SAII, for the rest of samples prepared with organic additive stacking degree slightly diminished respecting that found in either CMd or CMc formulations. SAI was the solid of the highest Mo4+ dispersion (DMoS2, from equation (5)) in full agreement with that previously reported (Escobar et al., 2018) where MoS2 slab length diminished in NiMo/alumina sulfided catalysts solids with saccharide addition. Interestingly, enhanced oxidic Mo dispersion in CAI and CAII as evidenced by hypsochromic shift in low-energy absorption edge in UV region (Figure 3) was preserved in corresponding sulfided solids.
Weakened MoS2-support interaction in SAII probably due to augmented amount of deposited carbon residua playing support-like role (Ge et al., 2014) resulted in longer slabs diminishing then Mo4+ dispersion (Table 3). CA-modified solids had similar MoS2 particles morphology each other. Improved molybdenum sulfide slabs decoration by Co provoked by organic addition could significantly contribute to shortened slabs formation (Pimerzin et al., 2017). By using chelating agents that retarded low-temperature cobalt sulfidation MoS2 slabs growth could be prevented. Promoter atoms could be fixed on MoS2 edges after stable Co-complexes thermal decomposition (Nikulshin et al., 2014) once corresponding slabs had been already formed. On the other hand, in SA-modified solids carbonaceous residua could act as spacers hindering sulfided phases sintering thus improving their dispersion (Escobar et al., 2018). Indeed, by thermal analysis it has been found that decomposition of blocking agents (carbonaceous deposits avoiding large clusters formation) and metal sulfidation processes could occur simultaneously explaining why non-complexing additives could delay promoters sulfidation thus enhancing CoMoS phase formation (Pimerzin et al., 2017). It is worth mentioning that decreased interaction between alumina carrier and deposited MoS2 due to mentioned carbonaceous deposits could favor formation of so-called Type II sites (Nikulshin et al., 2012) characterized by significantly augmented HDS activity as to that of conventional Type I ones (Topsøe, 2007). Finally, it should be also considered that Mo-blue formation in solids prepared with saccharose could produce organic acids (by oxidation of fructose and glucose from saccharose hydrolysis) that could play the aforementioned role of chelating agents as Co sulfiding retardant (Nicosia & Prins, 2005b).
SAI was the solid of the highest MoS2 phase aspect ratio (ArMoS2, equation (3), Table 3) due to having the shortest average slab length (Shimada et al., 2016) that also provoked the lowest (fe/fc)MoS2 = (Moe/Moc) ratio pointing out to enhanced number of corner sites in molybdenum sulfide slabs. Interestingly, it has been proposed that under HDS conditions, exposed metal atoms in corner sites could be resistant to inhibition by strongly adsorbed nitrogen-bearing compounds (Rangarajan et al., 2017). Thus, materials with augmented population of those sites (thus of smaller MoS2 particles) could indeed be more active when hydrotreating real feedstocks containing aforementioned nitrogenated species. Also, it has been reported that MoS2 particles morphology (slab length, stacking and aspect ratio) could play significant roles upon selectivity to different hydrotreating reactions (Fan et al., 2009).
Very interestingly sulfur-containing Mo-blue has been obtained by MoS2 nanotubes exfoliation (Visic et al., 2013) where S 2p3/2 and 2p1/2 XPS peaks where identified at 169.2 and 170.4 eV being assigned to 4+ or 6+ valence states which could be related to SO4- or SO3- type species. That fact suggested that after catalysts activation (see section 2.1. Catalysts synthesis) some of those S-containing Mo-blue species could exist in our sulfided materials. Deeper insights in this aspect are needed.
Finally, catalytic test (activity and selectivity) of prepared organic agents-modified sulfided materials in the guayacol HDO and correlation of those data with the determined textural, structural and surface properties are in due course and will be presented in upcoming report. Interestingly, very good activity in the dimethylsufide elimination through oxidative desulfurization to corresponding sulfoxide catalyzed by Mo-blue species has also been recently reported (Ratheshkumar et al., 2020). Also, Mo-blue has been applied as catalyst of enhanced activity and stability in the selective oxidation of cyclohexane to cyclohexanol and cyclohexanone (Liu et al., 2015). Catalysts containing “NiMoS” phases from alumina-supported Mo-blue precursors including partially reduced molybdenum species (Mo5+ entities) have shown very high activity in HDS reaction as well (Escobar et al., 2018).
4. Conclusions
Saccharose (SA) and citric acid (CA) were used as additives in P-doped CoMo/Al2O3 catalysts (Mo, Co and P at 12, 3, and 1.6 wt%, respectively). One-pot impregnating solutions were prepared by MoO3 digestion in aqueous H3PO4, followed by cobalt acetate addition. Organics were integrated (SA/Co=1, CA/Co=2) at two different preparation stages. During method I SA or CA was added in as-prepared Co-Mo-P one-pot impregnating solution, (pH~2) followed by pore-filling impregnation of alumina support whereas in method II prior to Co-Mo-P phases deposition SA or CA was impregnated on the pristine carrier, followed by drying (120 °C). Co-complexation by CA and Mo-blue (LMCT complex) formation (SA-modified solids) were identified by UV-vis analyses of corresponding oxidic dried samples. Also, partially reduced molybdenum species (Mo6+ => Mo5+, thus Mo-blue, by XPS) was observed after organics addition (either SA and CA) that effect being more evident in saccharose-modified solids. After sulfiding (H2S/H2 10%, 400 °C, 2 h) Co-Mo-P phases impregnation in one-pot solution simultaneously deposited with SA rendered the materials of the highest MoS2 dispersion (as determined by HR-TEM).
Conflict of interest
The authors have no conflict of interest to declare.
Financing
This investigation was funded through the D.061048 IMP grant.