Introduction
The increasing prevalence in hypertension and risk factors, such as coronary heart disease, stroke, and kidney disease, have recently received considerable attention. Nowadays, interest in using bioactive ingredients is the cornerstone of hypertension prevention (Patten et al., 2016; Li et al., 2019; Zhu et al., 2019). Blood pressure regulation, controlled by the renin-angiotensin-aldosterone system, is vital for preventing this disease. Angiotensin-converting enzyme (ACE) converts angiotensin I into the potent vasoconstrictor, angiotensin II, which elevates blood pressure by inducing vasoconstriction of the smooth muscle cells of the arteriole vasculature (Patten et al., 2016). Thus, ACE-I could be a target for inhibition to prevent hypertension in patients.
Among all bioactive components found in foods, bioactive peptides have attracted the most attention due to their potential health benefits. These peptides are residues of 2 to 20 amino acids (smaller than 10 kDa) and are frequently encrypted in food proteins from which they can be released through hydrolysis by digestive (pepsin and trypsin), microbial, or plant (proteinases and peptidases) enzymes (Cian et al., 2015). Some reports suggest that different bioactive peptides in diverse food proteins have the potential to inhibit the ACE-I (Cai et al., 2015; Zhu et al., 2019). Similarly, Li et al. (2019) reported that to date, 22 sequences of bioactive peptides have been identified from CGM, all of these could potentially be used in the treatment of hypertension and oxidative stress. Collectively, the search for compounds able to control/ prevent hypertension, such as protein-derived peptides from foods, represent a promising source for health-enhancing components (Patten et al., 2016; Li et al., 2019).
Corn (Zea mays L.) is a well-known grain, cultivated since ancient times and widely consumed around the world. Corn is used in different forms, the raw material for the elaboration of many products and the corn-derived products used for industrial applications (Da Silva-Messias et al., 2015). In Mexico exist at least 59 to 61 corn landraces. Among them, some pigmented landraces of colors such as yellow, black, red and blue, have been identified and approximately a third of the national producers harvest these corn varieties (Sánchez et al., 2000). In the state of Sinaloa, located in Northwest Mexico, the most important pigmented landrace is “Elotero de Sinaloa”, characterized by its blue color (Gaxiola-Cuevas et al., 2017). Uriarte-Aceves et al. (2015) worked with 15 Mexican blue corn genotypes, and reported that they possess characteristics that make them appropriate for wet-milling processing. Traditionally, corn wet-milling is a process that separates the corn in high purity fractions called by-products, such as corn gluten meal (CGM), which contains the greatest percentage of protein (61 to 62%), consisting mainly of 65 % zeins and 30 % glutelins (Anderson and Lamsal, 2011; Zheng et al., 2015). Despite corn proteins are deficient in lysine, histidine, and tryptophan (essential amino acids), thus affecting their nutritional quality, their overall amino acid profile, could make corn proteins a source of bioactive peptides (Zhu et al., 2019).
Some researchers have demonstrated the antioxidant properties (Li et al., 2008; Li et al., 2019) and anti-hypertensive benefits (Cai et al., 2015; Li et al., 2019) of white CGM. Thus, the blue CGM could also be a source of bioactive peptides with antioxidant and anti-hypertensive activities, due to its amino acid profile.
Blue corn consumption has been associated with health benefits, attributed to its high content of phenolic acids and anthocyanins (Gaxiola-Cuevas et al., 2017). Never-theless, just a few reports have studied the antioxidant activity and anti-hypertensive properties of the CGM peptides that could be released from the hydrolyzed protein of blue corn. This study aimed to demonstrate the in vitro and in vivo ACE inhibitory activity of CGM hydrolysates from blue corn.
Materials and methods
Corn sample
Blue creole corn (Zea mays L.) identified as “Elotero de Sinaloa” was collected from the municipality of Concordia, Sinaloa, Mexico. The grains were grown and harvested during the 2016 season. Crops were handled following standard recommendations, and corn samples were hand cleaned to remove broken kernels and foreign material, placed in plastic bags, and stored at 4°C until wet-milling process.
Proximate composition
The AOAC (1990) official methods were used to determinate the proximate composition of raw blue corn and blue CGM. Moisture was measured by drying the samples at 105 °C for 24 h (method 925.09B). For ash determination, the samples were incinerated at 550 °C (method 923.03). To evaluate crude fat, a Soxhlet apparatus with petroleum ether was used (method 920.39C), and for protein estimation, the micro-Kjeldahl method (N x 6.25) (method 960.52) was performed. All experiments were conducted by triplicate.
Blue corn wet-milling process
The method by Singh et al. (1997) using 100 g of corn and slightly modified by Taboada-Gaytan et al. (2009) was used. The procedure consists of 6 steps: 1) soak stage, 2) first milling, 3) germ recovery and coarse fiber, 4) second grinding, 5) fine fiber separation, and 6) separation of starch fraction and corn gluten meal (CGM). All fractions obtained were dried by the method 964.22 (AOAC, 1990). The yield percentage was calculated on a dry basis (db) for each fraction.
Preparation of blue CGM hydrolysates
In vitro simulated digestion of blue CGM was performed following the modified procedure by Montoya-Rodríguez et al. (2014). Briefly, blue CGM flour was suspended in water (1:20 w/v) and a sequential enzyme digestion was carried out with pepsin (EC 3.4.23.1, 662 units/mg; enzyme/ substrate, 1:20 w/w; pH 2.0) and pancreatin (EC 232-468-9, 8 x USP; enzyme/substrate, 1:20 w/w; pH 7.5). The solution was incubated at 37 °C at different times for each enzyme. The reaction was stopped by heating at 75 °C for 20 min, and the final hydrolysate was centrifuged at 20,000 × g for 15 min at 4 °C. To determinate the hydrolysis, aliquots of 50 mL were initially hydrolyzed during 180 min with pepsin and after that with pancreatin for additional 20, 50, 80, and 180 min. The hydrolysates were then frozen, lyophilized, and kept at -20 °C until their use.
Measurement of soluble protein
The total soluble protein of hydrolysates and blue CGM was determined by DCTM protein assay kit (Bio-Rad Laboratories, Hercules, CA). The amount of soluble protein was calculated using a standard calibration curve built by using different bovine serum albumin concentrations from 125 to 1250 µg/mL, in a PBS solution. The absorbance was measured in a spectrophotometer (GENESYS 20, Sigma Aldrich, USA) at 690 nm.
Measurement of antioxidant activity
The antioxidant activity of blue CGM hydrolysates was evaluated with the oxygen radical absorbance capacity (ORAC) method, as previously described by Ou et al. (2009). The assay was performed using fluorescein as the fluorescent probe, AAPH as the radical generating agent, and Trolox as the standard. Hydrolysates, Trolox and AAPH were diluted with sodium phosphate buffer (75mM, pH 7.4). Hydrolysate aliquots (25 µL) were transferred into a 96-well plate, loaded into the plate holder of the Synergy Microplate Reader (SynergyTM HT Multi-Detection, BioTek, Inc., Winooski, VT) for the kinetic reading. Results of the protective effects of the blue CGM hydrolysates and control were calculated based on the differences in the area under the sodium fluorescein decay curves (AUC) between the blank and the sample (Roy et al., 2010). The antioxidant activity of hydrolysates was expressed as µmol of Trolox equivalents (TE) per mg of soluble protein.
SDS-PAGE of blue CGM hydrolysates
Samples of blue CGM were diluted with Laemmli Sample Buffer (Bio-Rad Laboratories, Hercules, CA) with β-mercaptoethanol and boiled for 5 min before loading. The protein profile of the hydrolysates was analyzed by SDS-PAGE using 10 and 12 % gels by the method of Laemmli (1970). A pre-stained precision Plus Protein Standard Dual color (Bio-Rad) was used. The gel was run at 110 V for 95 min in solution 10 % Tris-Glycine Buffer and 90 % water. Then, the gel was stained with Simplyblue TM Safe Staining solution (Thermo-Fisher Scientific, MA, USA) (Coomassie Brilliant Blue R250, distilled water, methanol, glacial acetic acid, trichloroacetic acid) overnight. After that, it was washed-out with de-staining solution (distilled water, methanol, glacial acetic acid, trichloroacetic acid) once during 30 min. Finally, it was washed with distilled water.
Determination of percent inhibition of ACE-I activity
ACE inhibitory activity was carried out by the ACE Kit-WST assay kit (Lam et al., 2007). This assay is based in the action mechanism of the angiotensin-converting enzyme (ACE). This enzyme is a zinc-containing nonspecific dipeptidyl carboxypeptidase (EC 3.4.15.1), released mainly from endothelial cells of the capillaries of the lungs and kidneys, that converts angiotensin I to an active octapeptide vasoconstrictor, angiotensin II through the removal of two-terminal amino acid residues (Lam et al., 2007). The assay determines the amount of 3-hydroxybutyric acid produced and it is measured at 450 nm by spectrophotometric method. The inhibitory activity of the samples was calculated as follows and the results expressed as a percentage of ACE-I activity inhibition:
The inhibitory activity of ACE (% inhibition rate) = [(A Blank 1 - A sample) / (A Blank 1 - Blank 2)] X 100.
Evaluation of ACE-I inhibition in vivo
The Bioethics Committee approved the study protocol of the Autonomous University of Sinaloa (CE-UACNYG-2015-SEP-001). Female Balb/c mice (n=18, 11 weeks old, 25-30 g) were purchased from BioInvert, México City, México. All mice were housed in plastic cages with stainless steel lids in a room with controlled temperature (28 °C) and lighting (12-h light/dark cycle). Food (Rodent Lab Chow 5001) and water were available ad libitum. To determine the in vivo bioavailability of blue CGM peptides as well as their efficacy inhibiting ACE-I activity, mice were divided randomly into one of three groups. The first group (n=6) received the 260 min CGM hydrolysate (pepsin 180 + pancreatin 80 min) (2.5 g/kg of body weight) resuspended in water. The second group (n=6) received the ACE inhibitor Captopril (25 mg/kg of body weight) solubilized in water (positive control). The third group (n=6) received only PBS (300 µL) (negative control). All treatments were administered by intragastric gavage using plastic feeding tubes (20 GA x 38 mm, Instech Laboratories, Inc). Blood samples were drawn from the tail vein before and after 5, 10, 15, 30, and 60 min of the intragastric interventions. Blood samples were centrifuged at 2000 x g for 20 min at 24 °C to collect the serum (Ramírez-Torres et al., 2017). The presence of the antihypertensive compounds in the serum samples was determined using the ACE inhibition kit (ACE kit-WST-Dojindo Molecular Technologies, inc., Kumamoto, Japan) following the manufacturer´s instructions.
Results and discussion
Chemical composition
The chemical composition of raw blue corn and blue CGM varied significantly (p ≤ 0.05) (Table 1). The protein content in blue corn was 8.9 %. Our results are consistent to the protein values (7.6 to 11.2 %) reported in 15 blue corn genotypes from the state of Sinaloa (Uriarte-Aceves et al., 2015), but slightly lower to the values (11.95 %) reported by Taboada-Gaytan et al. (2009). On the other hand, after wet-milling, in the CGM we observed a 3.5-fold protein content increase (31.6% vs 8.9% in raw corn, p ≤ 0.05) compared to the raw material. During this process, the lactic acid used generates the hydrolysis of some corn molecules, mainly proteins (Uriarte-Aceves et al., 2015). The tabling method has been for years the most used technique for wet-milling, which separates the starch from the gluten meal fraction (Singh et al., 1997; Uriarte-Aceves et al., 2015).
Table 1 Chemical composition of blue corn and blue corn gluten meal Tabla 1. Composición química de maíz azul y harina de gluten de maíz azul
Properties | RBC (%) | CGM (%) |
---|---|---|
Proteins | 8.9±0.2 b1 | 31.6±1.6 a |
Lipids | 5.0±0.5 b | 13.6±0.4 a |
Ashes | 1.4±0.03 b | 6.9±0.03a |
Carbohydrates | 84.6±0.3 a | 47.9±1.6 b |
1Mean ± SD (n= 3). Means with different superscripts in the same row are significantly different (Tukey test, p< 0.05). RBC= raw blue corn. CGM= blue corn gluten meal.
The lipid content of the raw blue corn was 5.02 %. Uriarte-Aceves et al. (2015) reported lipid content values varying from 4.4 to 6.5 % in Mexican blue corn genotypes, while Taboada-Gaytan et al. (2009) have reported values slightly lower compared to this study. Compared to the raw material, a 2.72-fold increase (p ≤ 0.05) in the lipid content of CGM was observed (Table 1).
It is known that CGM has 4.8-fold high ash content compared with raw corn. The higher concentration of ash in CGM (6.9 vs 1.4 % in raw corn, p ≤ 0.05) could be attributed to the solids solubilization, such as minerals, during the soaking step of the wet-milling process (Serna-Saldívar, 1996). Overall, our results suggest that the wet-milling procedure is a practical approach for the isolation of corn gluten meal from blue corn genotypes.
Wet-milling characteristics
The blue corn products obtained during the wet-milling process (starch, gluten, fiber, germ and soaking solids) were expressed as a percent of dry kernel weight. In industry, starch is considered an indicator of millability (i.e. milling characteristics). Starch yield and recovery are the most critical parameters to determine millability among corn genotypes (Singh et al., 1997; Uriarte-Aceves et al., 2015).
In our study, the starch recovery obtained for blue corn was 48.7 % (Table 2). Although some authors have reported higher values of starch recovery (54.2 to 63.8 %) (Taboada-Gaytan et al., 2010; Uriarte-Aceves et al., 2015), these variations could be attributed to the physical properties and chemical composition of the corn (Vignaux et al., 2006). The gluten recovery was 7.1 %, similarly to the results reported by Milasinovic et al. (2007) (5.3-13.5 %), and Uriarte-Aceves et al. (2015) (6.9-9.5 %). In contrast, Taboada-Gaytan et al. (2010) reported higher gluten recovery values (15.5-20.3 %). In spite of that, our results were higher than the typical gluten yields obtained at the industry level (5 %) (Johnson and May, 2003). The overall poor release of starch and gluten can be explained by the high protein content in the corn kernel (Vignaux et al., 2006).
Table 2 Blue corn wet-milling performance
Tabla 2. Rendimiento de molienda húmeda de maíz
azul
Wet-milling fractions | DB % |
---|---|
Starch | 48.7 ± 2.01 |
Gluten | 7.1 ± 0.3 |
Fiber | 25.5 ± 1.0 |
Germ | 3.8 ± 0.3 |
Soaking solids | 2.9 ± 0.1 |
1Mean ± SD (n= 3). DB= Dry Basis
The fiber content obtained was 25.5 %, comparable to the values reported by Uriarte-Aceves et al. (2015) (14.5-25.7 %), and higher that the standard industrial fiber yield of 11.5% as mentioned by Johnson and May (2003). The germ recovery during the wet-milling process of blue corn was 3.8 %. Vignaoux et al. (2006) reported similar amounts of this fraction (4.6-7.0 %) using different scales of wet-milling procedures. However, the mean value found in this study is below than those published by the wet-milling industry (7.5 %).
Finally, the last by-product of the wet-milling process are the soaking solids. In the current study we found a mean of 2.9 %, this value was similar to the results reported by Uriarte-Aceves et al. (2015), although lower than those reported by Vignaoux et al. (2006) (7.6 %). These differences could be attributed to the use of different corn varieties.
Soluble protein
In Table 3 the solubility of blue corn gluten meal (CGM) proteins is shown. Initially, the soluble protein value for unhydrolyzed CGM was 3.9 mg/mL. However, the hydrolysates CGM sample had a significant (p ≤ 0.05) increase (up to 4-fold after pepsin and pancreatin hydrolysis) at different digestion times. These increments of protein solubility were observed after pepsin digestion of CGM at 180 min (11.2 mg/mL), with highest value of protein solubility (15.1 mg/ mL) observed after 360 min digestion. These results could be related to the fact that the protein solubility increases with the hydrolysis, as it is primarily the result of the reduction in molecular weight and the increase in the number of polar groups. Our results are comparable with data reported by other researchers (Montoya-Rodríguez et al., 2014).
Table 3 Soluble protein and antioxidant activity of blue corn gluten meal
Tabla
3. Proteína soluble y actividad antioxidante en harina de
gluten de maíz azul
Material | Pepsin/ Pancreatin Digestion Time (min) | Soluble Protein (mg/mL) | Antioxidant Activity (μmol of TE/ mg of soluble protein) |
---|---|---|---|
CGM (unhydrolyzed) | 0 | 3.9 ± 0.2c1 | 69.6 ± 2.2e |
CGM + Pepsin | 180 | 11.2 ± 1.5b | 241.2 ± 8.1d |
CGM + Pepsin 180 min + Pancreatin | 20 (200) | 11.2 ± 1.2b | 242.5 ± 4.3d |
50 (230) | 12.3 ± 0.4b | 387.2 ±35.5b | |
80 (260) | 13.1 ± 0.8ab | 336.3 ± 9.50c | |
180 (360) | 15.1 ± 0.4a | 560.1 ± 23.4a |
1Mean ± SD (n= 3). Means with different superscripts within columns are significantly different (Tukey test, p ≤ 0.05). CGM= blue corn gluten meal. TE = Trolox Equivalents.
Antioxidant activity
The hydrolysis process was essential to release compounds with antioxidant activity compared with the unhydrolyzed form of CGM (Table 3). Some reports (Montoya-Rodríguez et al., 2014), have explained that the antioxidant property increases (up to 8 times) as a function of the hydrolysis time (360 min) compared to the unhydrolyzed CGM (Table 3). The high antioxidant activity (560.1 µmol TE/mg soluble protein) of the hydrolysates could be explained, in part, to the structural changes of the corn proteins. Enzymatic hydrolysis promotes the unfolding and exposition of residues of active amino acids and availability to react with oxidants (Kong and Xiong, 2006). Cereals, like corn, have been reported to present a particular amino acid composition. They contain high amounts of hydrophobic amino acids such as leucine, alanine, and phenylalanine. Thus, CGM protein was thought to be an excellent source to obtain peptides with antioxidant activity (Li et al., 2008; Li et al., 2019). Also, low molecular weight peptides have often been associated with high anti-oxidant activity (Cai et al., 2015). Li et al. (2008) reported that the antioxidant activity of corn peptides is closely connected with their molecular weight. They suggested that corn peptides with a molecular weight between 0.5 to 1.5 kDa show higher antioxidant activity. Interestingly, Zhou et al. (2012) found variations in the antioxidant activity assessed by ORAC method between 0.066-0.19 µmol TE/ mg of dry weight in corn peptides of 1-10 kDa. Other authors report minor anti-oxidant activity in blue corn from Chiapas and hybrid white corn using the same method (0.81 and 1.055 µM TE/mg of peptide) in hydrolyzed zein with peptides from 20-25 kDa (Díaz-Gómez et al., 2018). However, in the results obtained in our work we observed than the antioxidant activity found in hidrolyzed CGM is higher (241.2-560 µmol TE/mg soluble protein) than that reported by other authors (Zhou et al., 2012; Díaz-Gómez et al., 2018). It is important to mention that the size of the protein or peptide fraction found in this study was between 19 to 31 kDa. However, fractions <10 kDa were also observed. Evidently, these results demonstrate important differences regarding the starting material (CGM vs corn protein and zein fractions), and that hydrolysis conditions could have an important effect on the size and sequence of the active peptide (Díaz-Gómez et al., 2018).
CGM protein profile
The SDS-PAGE analysis (Figure 1) was performed to assess the protein profile of the blue CGM hydrolysates. We noticed different protein profiles in the CGM, with proteins of different molecular weights ranging from 15 to 50 kDa. In Figure 1, it is shown that the protein profile changed during the hydrolysis time. Furthermore, at the end of hydrolysis, mainly lower molecular weight bands (peptides) are observed (< 10 kDa). Cai et al. (2015) described that corn peptides with a small molecular weight showed good anti-hypertensive activity in both, in vitro and in vivo assays. In addition, a permanent band corresponding to α-Zein (27 kDa) was observed after hydrolysis (360 min). This band is related to the corn content of glutamine and proline, amino acids with poor solubility and relative low surface/weight ratio (Cabrera-Chávez et al., 2012) . Zheng et al. (2015) reported that the main protein fractions in CGM consist of approximately 65-68% (w/w) zeins and 22-33 % glutelins (w/w). These reports are in agreement with the results of this work.
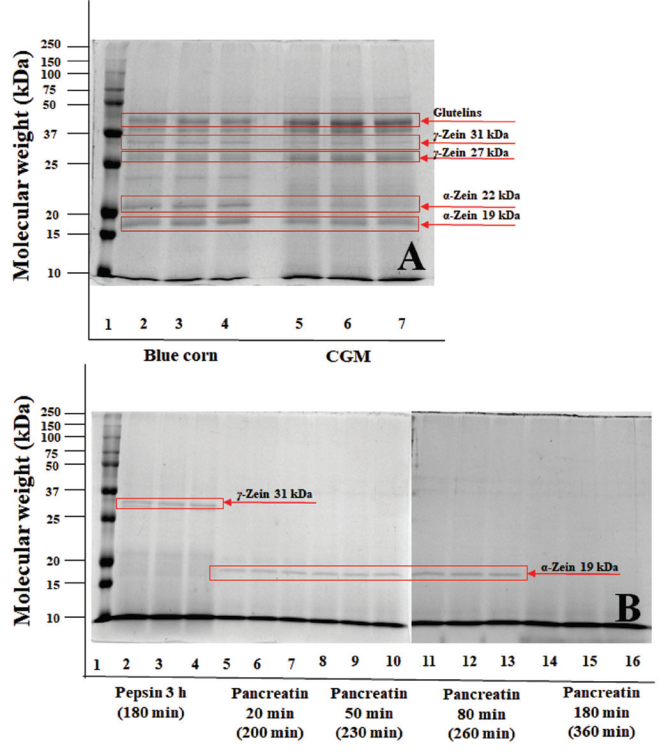
Figure 1 SDS-PAGE of blue corn, blue CGM, and blue CGM hydrolysates at different times: A)
Standard lane 1, lane 2-4 blue corn, lane 5-7 blue corn gluten meal;
B) Standard lane 1, lane 2-4 blue CGM hydrolyzed with pepsin for 180
min, lane 5-7 blue CGM hydrolyzed with pepsin for 180 min +
pancreatin 20 min (200 min), lane 8-10 blue CGM hydrolyzed with
pepsin for 180 min + pancreatin 50 min (230 min), lane 11-13 blue
CGM hydrolyzed with pepsin for 180 min + pancreatin 80 min (260
min), lane 14-16 blue CGM hydrolyzed with pepsin for 180 min +
pancreatin 180 min (360 min)
Figura 1. SDS-PAGE
de maíz azul, HGM azul, e hidrolizados de HGM azul a diferentes
tiempos: A) Línea estándar 1, línea 2-4 maíz azul, línea 5-7 harina
de gluten de maíz azul ; B) Línea estándar 1, línea 2-4 HGM azul
hidrolizado con pepsina por 180 minutos, línea 5-7 HGM azul
hidrolizado con pepsina por 180 minutos + 20 minutos con pancreatina
(200 minutos), línea 8-10 HGM azul hidrolizado con pepsina por 180
minutos + 50 minutos con pancreatina (230 minutos), línea 11-13 HGM
azul hidrolizado con pepsina por 180 minutos + 80 minutos con
pancreatina (260 minutos), línea 14-16 HGM azul hidrolizado con
pepsina por 180 minutos + 180 minutos con pancreatina (360
minutos).
Inhibition of ACE-I activity
The results of angiotensin-converting enzyme (ACE) activity inhibition are presented as the percent of inhibition of the ACE obtained by the blue CGM after enzymatic digestion with pepsin for 180 min and after with pancreatin at different times (hydrolysates). Undigested (unhydrolyzed) CGM (time = 0) exhibited the highest (96.3 %) ACE-I inhibitory activity compared to all CGM-hydrolysates (89.7 to 92.9% inhibition) (Table 4). The 200 min hydrolysate (Pepsin 180 min + Pancreatin for 20 min) showed the highest percentage of ACE-I inhibition (94.5 %), while the lowest inhibition value (89.7 %) was exhibited after 360 min digestion (Pepsin 180 min + Pancreatin 180 min). These results suggest that the digestion of blue CGM with pancreatin after 180 minutes of pepsin increases its ACE-I inhibitory activity, however digestion with pancreatin should not be longer than 80 minutes (i.e. 260 min digestion), because after this time, the ACE-I inhibitory activity starts to decrease (Table 4). Thus the 260 min CGM hydrolysate will be use for the in vivo studies.
Table 4 ACE activity inhibition by blue CGM Tabla 4. Inhibición de ECA por hidrolizados de maíz azul
Material | Pepsin/ Pancreatin Digestion Time (min) | ACE Activity Inhibition (%) |
---|---|---|
CGM (unhydrolyzed) | 0 | 96.3 ± 0.1a1 |
CGM+ Pepsin | 180 | 92.9 ± 0.3c |
CGM + pepsin 180 min + pancreatin | 20 (200) | 94.5 ± 0.4b |
50 (230) | 93.7 ± 0.6bc | |
80 (260) | 94.3 ± 0.1b | |
180 (360) | 89.7 ± 0.0d |
1Mean ± SD (n= 3). Means with different superscripts within columns are significantly different (Tukey test, p ≤ 0.05). CGM= blue corn gluten meal. ACE = Angiotensin-converting enzyme.
García et al. (2013) reported that the ACE inhibitor-peptides could only exert their antihypertensive potential if they reach the cardiovascular system in their active form. After oral ingestion, they need to resist gastrointestinal digestion by proteases and peptidases. Peptide degradation could occur during this process, with consequent inactivation. Unexpectedly, in this investigation we found that the unhydrolyzed CGM had higher ACE-I inhibitory activity than the hydrolyzed CGM at any time. It is essential to mention that several conditions contribute to the composition and functional activities of bioactive peptides. Such as enzyme specificity, degree of hydrolysis, enzyme/substrate ratio, hydrolysis time, and amino acid sequence (Zhu et al., 2019).
Determination of bioavailability
The bioavailability of the antihypertensive biopeptides was assessed in vivo in BALB/c mice. The CGM peptides capacity to reach circulation and had an effect on ACE-I activity was indirectly evaluated using the ACE-inhibition kit previously mentioned. Figure 2 shows the percentage of ACE-I activity inhibition at different time points after treating the mice with either blue CGM hydrolysate, captopril, or PBS solution. In this study, the PBS group showed an ACE-I inhibitory effect of 29 % at 5 min that started progressively decreasing after that until it reached basal levels after 15 min. Amir et al. (1980) explained that when mice suffer stress by an intragastric supplementation, enkephalins (considered a neuropeptide) are released, and these polypeptides could act as substrates for/or ACE-I inhibitors. The blue CGM hydrolysate was detected in the circulation after 5 min and its levels were maintained for up to 30 min post-supplementation. The bioavailability of the hydrolysate supplement was similar to that observed for the ACE-inhibitor drug captopril, whose levels were observed in serum at 5 min and maintained for up to 1h after administration. In the CGM hydrolysate group, the highest ACE-inhibitory activity was observed from 5 to 15 min after ingestion (up to 59 % inhibition), and these values were significantly different (p ≤ 0.05) to the PBS group. The hydrolysate group decreased its inhibitory activity by ~44 % after 30 min and reached basal levels at 60 min (0 % inhibition) after treatment.

Figure 2 Bioavailability of blue CGM hydrolysate and ACE inhibitory capacity. Serum from mice supplemented with PBS solution (0.3 mL, n=6, negative control), blue CGM hydrolysates (260 min digestion) (2.5 g/kg, n=6), and captopril (25 mg/kg, n=6, positive control), were utilized for the assays. PBS (line blue), blue CGM hydrolysates (orange line), and captopril (gray line). Capital letters indicate differences across time points for each treatment (PBS, hydrolysate, and captopril). Lowercase letters indicate differences among treatments (PBS, hydrolysate, and captopril) at the same time point
The maximum ACE inhibitory activity in the captopril group started at 5 min and was maintained for up to 15 min after ingestion (up to 54.6 %, which was normalized to 100 % inhibition). Although the captopril showed a decrement of 72 % in its activity after 60 min, it did not reach basal levels.
In agreement with our data, early studies have reported that in murine models, the maximum ACE percent inhibition of captopril in the blood is reached within the first 20 min post-supplementation (Popovici et al., 2011; Ramirez-Torres et al., 2017). Guo et al. (2019), demonstrated that the mixture of peptides derived from the CGM showed good long-term anti-hypertensive effect during 35 days in spontaneously hypertensive rats. The same authors also reported that high-dose of intragastrically administered CGM reduced the systolic blood pressure of rats from 18.2 to 28.6 mmHg (57 to 81 % reduction).
Collectively, our results suggest that CGM hydrolysates have the potential to inhibit ACE in vivo after the bioactive peptides reach the bloodstream. The positive effect was detected after 5 min of intragastric administration. Sánchez-Rivera et al. (2014), reported that the anti-hypertensive peptides could be detected in blood after 5 min post-intragastric-challenge and reach a maximum level after 30 min of administration. In our study, serum from mice treated with the CGM hydrolysate obtained 30 min post-intragastric-challenge was still able to inhibit 44 % of the ACE activity.
Conclusions
The wet-milling process generates high quality by-products as gluten, starch, fiber, and germ present in blue corn. The obtained CGM showed high protein content and peptides released by simulated gastrointestinal digestion which showed high antioxidant activity. Furthermore, the CGM hydrolysate showed a maximum ACE-I inhibition of 59% and was bioavailable in mice from 5 to 30 min of administration, similar to the pattern observed with captopril.
Finally, our results suggest that consumption of blue CGM hydrolysates could serve as supplements or functional ingredients for the development of new foods, since they are a source of peptides with anti-hypertensive properties. Although, this research showed a positive effect of ACE-I inhibition with CGM hydrolysates, it is necessary to further determine the long-term effect of the hydrolysates in spontaneously hypertensive rats, monitoring their blood pressure after intragastric interventions at different time points.