Serviços Personalizados
Journal
Artigo
Indicadores
-
Citado por SciELO
-
Acessos
Links relacionados
-
Similares em SciELO
Compartilhar
Agrociencia
versão On-line ISSN 2521-9766versão impressa ISSN 1405-3195
Agrociencia vol.50 no.2 Texcoco Fev./Mar. 2016
Natural renewable resources
Organic carbon content and water retention in soils of a cloud forest in Michoacán, México
1 Escuela Nacional de Estudios Superiores, Unidad Morelia. Antigua Carretera a Pátzcuaro Núm. 8701, Colonia ExHacienda de San José de la Huerta, 58190 Morelia, Michoacán, México (carlosanaya.m@gmail.com).
2 Centro de Investigaciones en Geografía Ambiental, Universidad Nacional Autónoma de México. Antigua Carretera a Pátzcuaro Núm. 8701, Colonia ExHacienda de San José de la Huerta, 58190 Morelia, Michoacán, México.
3 Facultad de Biología, Universidad Michoacana de San Nicolás de Hidalgo. Edificio “R”, Ciudad Universitaria, Morelia, Michoacán, México.
Cloud Forests (CFs) in Michoacan, Mexico, face strong pressures due to logging and the change of land use to agriculture, which can affect the ecological functions of the soil. This study identifies the main soils of a CF in Michoacan, and evaluates its contensts of Soil Organic Carbon (SOC) and water-retention capacity. The sampling design was stratified, considering different slope positions. Eight profiles of soils of 1 m in depth were characterized using standard methods. The soil groups identified, Andosol, Alisol, Umbrisol, displayed high degrees of weathering, high to neutral acidities, and a low saturation of bases. The SOC content fluctuated between 92 and 152 Mg ha-1; 55 % of this was distributed in the first 30 cm, and no differences were observed between soil groups. Water retention at high tension (HT, 1500 kPa) and low tension (LT, 30 kPa) varied from 98 to 284 L m-2, and from 249 to 510 L m-2, respectively. At a depth of 60 cm, where fine roots concentrate, the available water (AW) fluctuated between 107 and 167 L m-2, with no differences between soil groups. Water retention at HT had a negative correlation with the proportion of sands and the bulk density, whereas LT had a positive correlation with the concentration of SOC, and a negative one with bulk density. The AW had a positive correlation with the SOC concentration. The study confirms the importance of the CF in the storage of SOC and indicates that the SOC is determinant in the availability of water. Given the accumulation of SOC on the soil surface, carbon and water retention can be very sensitive to anthropogenic disturbances.
Keywords: Mountain cloud forest; soil organic carbon; soil moisture; ecosystem services; Trans-Mexican Volcanic Belt
Los bosques de niebla (BN) de Michoacán, México, enfrentan fuertes presiones de extracción forestal y cambio de uso de suelo a agricultura, las cuales pueden afectar las funciones ecológicas de los suelos. En este estudio se identificaron los principales suelos de un BN en Michoacán, y se evaluaron sus contenidos de carbono orgánico (COS) y capacidad de retención de agua. El diseño del muestreo fue estratificado, considerando diferentes posiciones de ladera. Ocho perfiles de suelo de 1 m de profundidad se caracterizaron con métodos estándar. Los grupos de suelo identificados, Andosol, Alisol, Umbrisol, mostraron un grado de intemperismo relativamente alto, acidez de alta a neutra y saturación de bases baja. El contenido de COS osciló de 92 a 152 Mg ha-1, el 55 % se distribuyó en los primeros 30 cm, y no hubo diferencias de contenido entre grupos de suelo. La retención de agua a tensión alta (TA, 1500 kPa) y baja (TB, 30 kPa) varió de 98 a 284 L m-2 y de 249 a 510 L m-2, respectivamente. A 60 cm de profundidad, donde las raíces finas se concentran, el agua disponible (AD) osciló de 107 a 167 L m-2, sin diferencias entre grupos de suelos. La retención de agua a TA tuvo correlación negativa con la proporción de arenas y la densidad aparente, mientras que a TB tuvo correlación positiva con la concentración de COS y negativa con la densidad aparente. El AD tuvo correlación positiva con la concentración de COS. El estudio confirma la importancia del BN en el almacenamiento de COS, e indica que el COS es determinante en la disponibilidad de agua. Dada la acumulación de COS en el suelo superficial, la retención de carbono y agua pueden ser muy sensibles a las perturbaciones antropogénicas.
Palabras clave: Bosque mesófilo de montaña; carbono orgánico del suelo; humedad del suelo; servicios ecosistémicos; Sistema Neovolcánico Transversal
Introduction
Cloud forests (CFs) or montane wet temperate forest cover approximately 1 % of Mexico’s territory (8800 km2; INEGI, 2007). Despite this small surface, CFs are considered biologically and environmentally very important for the country, since they harbor a large biodiversity and play a predominant part in the water, carbon, and nutrient biogeochemical cycles (CONABIO, 2010). Paradoxically, this biome faces a strong pressure due to logging and extraction of other resources, as well as the change of land use to agriculture, which affects its capacity to provide ecosystem services (Martínez et al., 2009). For example, agricultural fields, pasturelands, coffee plantations, and other land uses have replaced over 50 % of the original surface of the CF (CONABIO, 2010; Toledo-Aceves et al., 2011). Given this outlook, there is a need to increase our knowledge on environmental aspects of this biome in order to understand how it works and propose options for its sustainable use or justify its conservation.
The CF is defined by its location in mountainous regions and by the persistent or frequent presence of clouds at a canopy level throughout the year (Hamilton et al., 1995). In Mexico, this biome is distributed in a discontinuous manner in the Pacific and Atlantic watersheds and in the Trans-Mexican Volcanic Belt (TMVB), at altitudes of 1200 to 2500 masl, with temperate climates, and humid and sub-humid tropical climates (CONABIO, 2010). The knowledge about the CF is relatively broad in terms of its structure and floristic composition (Rzedowski, 1996; CONABIO, 2010; Villaseñor, 2010; González-Espinosa et al., 2011), although it is meager in terms of soil and other components. This deficiency has limited the possibility of having a more comprehensive vision of the CF.
Soil is a crucial component in the ecosystems that provides support to plants, it provides habitats for a large diversity of organisms, and is a subsystem where several process take place, all related to the flows of matter and energy. As a result of these processes, soil provides a series of vital ecosystemic services for the development of life on the planet, such as the regulation of the water cycle and carbon sequestration (Palm et al., 2007). For example, the carbon stored in the first meter of soil depth is known to duplicate the C content in the atmosphere, and that the reduction of this storage due to human activities is a source of CO2 for the atmosphere that can intensify the greenhouse effect, and consequently, climate change (Prentice et al., 2001).
Soil development depends on the conjugation of different characteristics of the locations, including the parent material, the relief, climate, vegetation, and time (Binkley and Fisher, 2013). Given the humid or sub-humid climate and the forest vegetation, CF soils may display common characteristics between them. For example, given the conditions of high humidity in the atmosphere and the low evapotranspiration, CF soils are influenced by the abundance of humidity throughout the year, which promotes high rates of chemical weathering of the mineral fraction and the development of anaerobic conditions that bring about acidic soils with a high accumulation of organic matter (Bruijnzeel and Proctor, 1995; Kitayarna, 1995; Benner et al., 2010). However, these forests also tend to display a considerable spatial heterogeneity in the characteristics of their soils, due to their location in mountainous regions with heterogeneous reliefs (Cotler, 2003). In a catena, for example, soils display variations in their depths, textures, and rockiness related to the relief (Birkeland, 1984), which condition water retention (Saxton and Rawls, 2006) and organic matter (Amundson, 2001; Lützow et al., 2006), as well as the aptitude for their uses (Cotler, 2003).
In Michoacan, Mexico, the CF is located mainly on the TMVB, in isolated fragments, covering ravines and hillsides where humidity in the air concentrates (CONABIO, 2010; Santana et al., 2014). These forests face strong pressure due to logging and changes in land use use to avocado plantations, which are leading to their degradation and loss of surface (CONABIO, 2010; Mendoza et al., 2011; Bravo-Espinosa et al., 2012). Given this scenario, there is a need to study the remnant forest fragments to analyze their land use aptitudes and evaluate the environmental consequences of their conservation or transformation. The aim of this study was to determine the content of carbon in, and the water retention of, a CF in the central section of the TMVB in Michoacan. Likewise, due to the lack of information on the soil for the forest studied, soils were classified at a second level in scarce detail.
Materials and Methods
Study site
The study was carried out in a CF located in the municipal areas of Villa Madero and Tacámbaro, Michoacan, between March and June 2013. This forest covers an area of approximately 16.6 km2 (INEGI, 2007) and is located in the central area of the TMVB, coordinated 19° 22’ and 19° 16’ N and 101° 19’ and 101° 23W (Figure 1). The area is part of a group of volcanic domes and cones with a large depression towards the South, towards the depression of the river Balsas, caused by the collapse of a caldera (Garduño-Monroy et al., 1999). The volcanic structures of the area form a range with irregular slopes of medium (10-15°) to high steepness 40-70° (Garduño-Monroy et al., 1999). The lithology has an intermediate composition (dacitic-andesitic) resulting from the previous volcanism, and after the formation of the caldera, in the middle and late Miocene (Gómez-Tuena, et al., 2005). According to INEGI (2007), the predominant soil group in the area is Acrisol. The climate is temperate sub humid with summer rainfall. Weather stations in Tacambaro (No. 16123) and Villa Madero (No. 16140) from the IMTA database (2013), located 10 km from the area studied, record average annual rainfalls and temperatures (1960-2010) of 1153 and 1194 mm, and 18.5 and 15.8 °C, respectively.
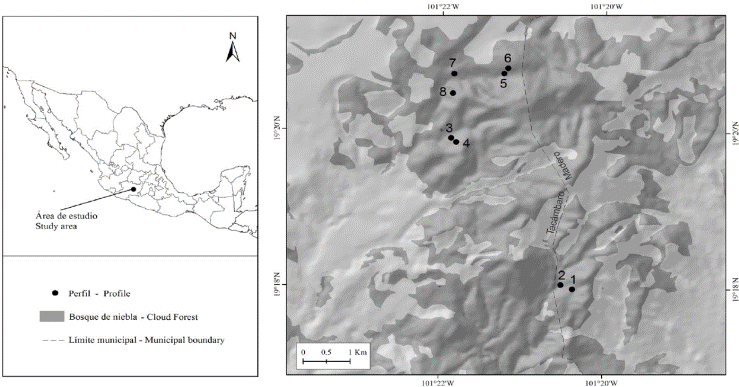
Figure 1 Location of the cloud forest studied. Dots indicate the location of the soil profiles and their labels.
In the region, the CF is found between 2000 and 2650 masl, in ravines and hillsides where humidity concentrates, surrounded by pine and pine-oak forests (CONABIO, 2010; Santana et al., 2014). Along with natural fragmentation, the CF displays a fragmentation related to the change in land use to agriculture, particularly avocado plantations (Mendoza et al., 2011; Santana et al., 2014). The forested areas display a certain degree of disturbance related to illegal selective logging and the extraction of resin. Some of the most abundant tree species include Alnus acuminata Kunth, Clethra mexicana DC., Quercus laurina Bonpl., Quercus rugosa Née, and Styrax argenteus (C. Presl.) Miers (Santana et al., 2014).
Soil sampling
Eight sites were chosen in the area of study, which were distributed into four sampling areas according to the possibilities of accessing the forest (including the consent of owners). Inside each area, two sampling sites were selected, located in different positions of the slope: high, medium, low, or foot (Table 1), geoforms in which the CF predominates. In each one, eight surface litter samples were taken from circular areas, each one 25 cm in diameter, and a one meter-deep test pit was dug (or until reaching a limiting layer or limiting material) for a soil profile description and the extraction of samples by horizon. A depth of 1 m was chosen, since this is the depth in which the highest concentration of organic carbon is found (Amundson, 2001) and in which water retention is most commonly studied and reported (Batjes, 1996; Malone et al., 2009). For each profile a routine description was performed (FAO, 2009) with standard methods (Siebe et al., 2006). For each soil horizon, the following were determined on the spot: 1) pH, using a Spectrum IQ-150 pH meter; 2) stoniness, estimated using reference charts; 3) presence of carbonates, found with the effervescence due to the reaction with HCl at 10 %; and 4) presence of active aluminum, determined by the reaction with sodium fluoride (NaF) 1N using phenolphthalein at 1 % as an indicator. A 2 kg sample was taken from each soil layer to determine its physical and chemical properties in the laboratory. Likewise, to determine the bulk density, two unaltered soil cores were taken from each layer using 40 cm3 rings.
Lab analysis
The soil cores were oven dried at 105 °C, until a constant weight was obtained. The bulk density (AD) was calculated by dividing the soil weight by the core volume; values were corrected by the weight and volume of the gravels (Elliott et al., 1999). The soil samples were dried at room temperature in the laboratory, sieved using a 2 mm sieve to obtain the fine fraction of the soil, and stored at room temperature for its physical and chemicals analysis. The soil texture was determined using the Bouyoucos Method, after eliminating organic matter (Gee and Bauder, 1986). Electric conductivity was determined in soil extracts using distilled water at a ratio of 1:5 (ISO 11265, 1994), using a conductivity meter (Oakton 8CU28). Phosphorous retention (PR) was determined using the Blakemore method (ISRIC-FAO, 2002), using a spectrophotometer (Jenway 6505 UV/Vis) to determine phosphorous concentrations. Humidity retention was determined using the pressure plate method (Klute, 1986). The water retention gravimetric percentages were determined at tensions of 30 kPa (field capacity; FC) and 1500 kPa (wilting point; WP). The available water (AW) was calculated as the difference between FC and WP (Osman, 2013). The water content in each soil layer at FC and WP was determined by multiplying the percentage of water in the soil at FC or WP with the quotient of the apparent soil density by the density of pure water (1 g cm-3) by the thickness of the soil layer (Osman, 2013). The values were adjusted due to the stoniness. The amount of water of the profile (in L m-2) was determined with the sum of the amounts of water in each layer. The cation-exchange capacity (CEC) was determined by the sodium saturation method (EPA 9081). This method tends to underestimate the CEC in comparison to the more accurate ammonium acetate method (Chapman, 1965). To determine the concentration of sodium, a flame photometer (Jenway PFP- 7) was used. The concentrations of interchangeable bases (Ca2+, Mg2+, K+, and Na+) were determined by ionic chromatography in a chromatograph (Metrohm Basic 883 IC-plus). Al and Fe were extracted with ammonium oxalate for 4 h in the dark at a pH of 3. The concentrations of Alo and Feo (i.e. Al and Fe in oxalate) were measured in an atomic absorption spectrometer (SpectrAA 220 Varian). The concentration of soil organic carbon (SOC) was determined by coulometry (Huffman, 1977) in a UIC CM 5012 total carbon analyzer. The content of SOC (Mg ha-1) in each horizon was determined by multiplying the concentration of C, by the bulk density, by the thickness of the horizon, by a factor of correction of the concentration of stoniness (1- fraction of stones), and by a spatial conversion unit (Amundson, 2001). The total amount of SOC in the soil profile was determined with the sum of the amounts of C of its layers.
The litter samples were oven dried at 70 °C for 72 h and weighed. The mass of litter per unit area was determined by dividing the mass by the area sampled. All analyses were performed in the Laboratorio de Suelo y Agua del Centro de Investigaciones en Geografía Ambiental, UNAM, except for the determinations of total C, which were carried out in the Laboratorio de Biogeoquímica of the Centro de Investigaciones en Ecosistemas, UNAM, and the determinations of Alo and Feo, which were carried out in the Laboratorio de Fertilidad de Suelos y Química Ambiental of the Colegio de Posgraduados.
Soil classification
Soils were classified according to the World Reference Base for Soil Resources (IUSS Working Group WRB, 2014). The classification was carried out at a second level, including the principal and supplementary qualifiers that apply according to the soil group, and for which there was certainty from the routine description and the physical and chemical analyses performed, omitting the qualifiers for which there was no data. For the classification of the Andosols, we used the values of Alo and Feo for horizons that, together, added up to over 30 cm.
Statistical analyses
To describe the changes in concentrations and contents of SOC based on the soil depth, the four regression models proposed by Jobbágy and Jackson (2000) were used: 1) base logarithm 10 (log) of the average depth of the horizon against the concentration of its SOC; 2) log of the average depth of the horizon against the log of its SOC concentration; 3) log of the horizon depth against the accumulated content of SOC; and 4) log of the depth of the horizon against the log of the accumulated content of SOC. These models assume that the relation between the depth with the concentrations and contents of SOC is not linear, and therefore the data are transformed logarithmically (Jobbágy and Jackson, 2000; Zar, 1999). The relation between the depth and the accumulated content of SOC has the advantage of the SOC storage being integrated from the superficial layer of the soil towards deeper layers. However, it has the statistical disadvantage of lacking independence between horizons for a non-linear adjustment (Jobbágy and Jackson, 2000).
In order to describe the effects of the bulk density, the percentages of clays, limes, sands, and SOC’s the on the FC, WP, and the AW, stepwise multiple regression analyses were performed (Gupta and Larson, 1979). Before the regression analysis, Pearson correlation analyses were carried out, and only the soil variables that had a significant correlation with the moisture conditions were included in the analysis (Zar, 1999). In the final model, the only independent variables included were those which had a significant effect on the humidity levels of the soils (Beta significant; β≠0) (Zar, 1999). These analyses required the use of the set of data from all eight profiles (n = 37). The statistical analyses were carried out using the STATISTICA 7.1 (Hill and Lewicki, 2007).
Results and Discussion
Soil classification
The soil profile characterization indicates the presence of three groups in the area under study: Andosol, Alisol, and Umbrisol (Table 2). The profiles 3, 5, 7, and 8 were classified in the group of Andosols, and had in common the Dystric qualifier (Table 2). There were no analyses on aluminum extracted with pyrophosphate to differentiate the Silandic and Aluandic qualifiers, although the presence of clear colors and the lack of extremely acidic pH values in all horizons with andic properties suggest the possibility of the Silandic qualifier (IUSS Working Group WRB, 2014). The Silandic and Aluandic qualifiers indicate differences in the quotients Al/ Si, as well as in the stabilization of organic matter due to the formation of organomineral complexes (Shoji et al., 1993; Campos-Cascaredo, 2000). The concentrations of Alo and Feo fluctuated from 2.1 % to 6.1 % and from 0.2 % to 1.2 %, respectively. These concentrations are considered high, which supports the inference of the Silandic qualifier, since they are indicative of high amounts of allophane (Parfitt and Clayden, 1991, Shoji et al., 1985). For example, Parfitt and Clayden (1991) show that an Alo concentration of 2 % is equal to nearly 8 % of allophane. The concentration of active Al (e.g. allophanes, imogolite) is implied in the adsorption of phosphorous, so the higher the concentration of active Al, the higher the phosphorous retention will be (Shoji et al., 1985). In our study, the Andosols displayed a phosphorous retention of over 88 % in its horizons with andic properties (Table 2).
Table 2 Physical and chemical characteristics of the soil profiles for a cloud forest in Michoacan, Mexico.
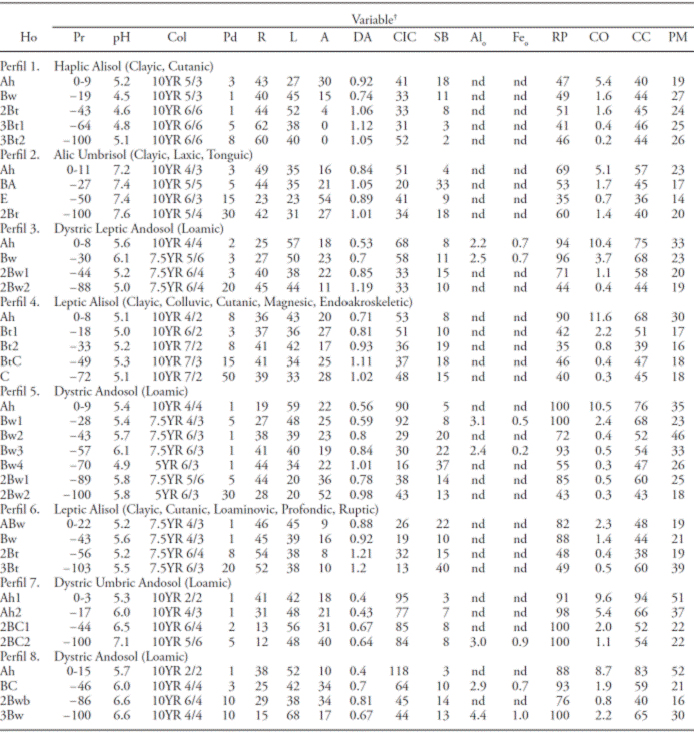
†Ho: Horizon; Pr: depth (cm); Col: color when dry; Pd: stoniness (%); R: clays (%); L: limes (%); A: sands(%); DA: bulk density (g cm-3); CIC: cationic exchange capacity (Cmol kg-1); SB: base saturation (%); Alo: aluminum in oxalate (%); Feo: iron in oxalate (%); PR: phosphorous (%); CO: organic carbon (%); FC: moisture retention at 30 kPa (%); WP: moisture retention at 1500 kPa (%); nd: not determined.
Due to its physical and chemical properties and the clay illuviation as a pedogenetic process, profiles 1, 4, and 6 were classified in the Alisol group (Table 2). These soils had a high PR, an acidic pH, and a low saturation of bases, which suggests the presence of minerals rich in aluminum (Shoji et al., 1996). In this case, these soils could be classified as Alumic, although this qualifier must be validated with the corresponding analyses.
Profile 2 was classified within the Umbrisol group (Table 2). Despite having all the processes and characteristics of the profiles classified within the Alisol group, this soil has an umbric horizon developed enough to be included in this group (IUSS Working Group WRB, 2014). Although the pH is not a criterion to differentiate between groups, it is interesting to notice that this soil had neutral pH values, unlike those in the Alisol group, which were more acidic.
The soils found are typical of mountainous regions with moderate to high moisture levels, and relatively high levels of weathering (IUSS Working Group WRB, 2014). These soils displayed certain chemical similarities amongst them, such a high to neutral acidity, low base saturation, and high contents of organic matter, which are characteristics that have been identified in CFs of other regions (Bruijnzeel and Proctor, 1995; Kitayarna, 1995). However, the presence of three different soil groups also denotes important soil heterogeneity in the studied area. This heterogeneity could be related to the action of different environmental conditions, such as topographic position, the soil’s humidity regime, and different development times, which determine the soil-formation processes, such as erosion, soil accumulation, clay illuviation and the rate of weathering of the parent material (Binkley and Fisher, 2013).
The INEGI (2007) indicates only the Acrisol group in the study area, in contrast to our results. This may be due, in part, to INEGI taking samples in a much lower resolution than in our study. In this way, our results complement the information of the state on soil, and may help improve its cartographic representation.
Organic carbon content
No carbonates were found using the reaction with HCl in any of the soils (data not shown). The content of SOC varied among profiles between 92 and 152 Mg C ha-1, with an average of 118 ± 7 Mg C ha-1 (Table 3). These values are in the lower section of the intervals reported for cloud forests in Chiapas (102 to 461 Mg C ha-1) and Oaxaca (158 to 222 Mg C ha-1) by De Jong et al. (1999) and Álvarez-Arteaga et al. (2013), respectively. However, those studies do not report that the contents of SOC were adjusted by the percentage of stoniness in the layers of soil; therefore their values may be overestimated. Our values lie within the interval reported for Andosols in undisturbed forests of fir (115 to 207 Mg C ha-1) and pine-oak (70 to 136 Mg C ha-1), of the Monarch Butterfly Biosphere Reserve (Pérez-Ramírez et al., 2013).
Table 3 Soil organic carbon content (SOC), water content at 30 kPa (FC), water content at 1500 kPa (WP), and available water (AD) by soil profile.
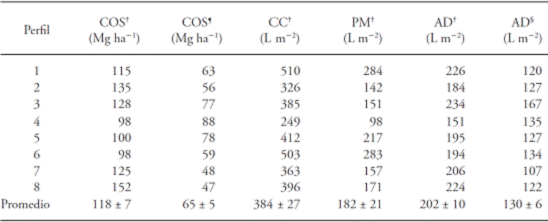
†Content at total depth of profile (see Table 2). ¶Content up to depth of 30 cm. §Content up to depth of 60 cm.
In all the profiles, the concentration of C was higher in the superficial layer of the soil, and decreased with depth (Table 2). On the other hand, the accumulated SOC (Mg C ha-1) naturally increased with depth (data not shown). Both behaviors were confirmed using the regression models, with a negative relation between depth and the content of SOC, and a positive relation between the depth and the accumulated SOC (Table 4). The best adjusted models were the log-log, which accounted for 78 % (R2=0.78) of the variation in the concentrations and contents of SOC with depth (Table 4). The significant adjustment of these models indicates that the relation between these variables is not linear, i.e. the magnitude of the changes in concentration and contents of SOC between layers decreases with depth. The highest concentration of SOC in the superficial layer of the soil is related to a higher incorporation of organic matter coming from the decomposition of surface litter and the turnover of fine roots in this layer, in comparison to deeper roots (Jobbagy and Jackson, 2000). However, the mechanisms that control the vertical distribution of organic matter in the soil profile are not yet fully understood; furthermore, these mechanisms may differ among soils (Jobbagy and Jackson, 2000; Rasse et al., 2005; Rumpel and Kögel-Knabner, 2011).
Table 4 Regression models that describe the variation in the concentration of Soil Organic Carbon (SOC) or the content of organic carbon ([SOC]) in function of the depth (Pr) in eight soil profiles in a cloud forest.

On average, 55 % of the total SOC content in the profiles was concentrated in the top 30 cm of soil (Table 3). Similarly, in an analysis of 1017 soil profiles in forests of the United States, Jobbagy and Jackson (2000) found that 50 % of the SOC was concentrated in the top 20 cm in profiles one meter deep. The superficial layer of the soil (< 30 cm) is very sensitive to direct or indirect human disturbances, such as deforestation and changes in land uses to agricultural systems (García-Oliva et al., 1999; Guo and Gifford, 2002; Don et al., 2011). Therefore, an implication of the high accumulation of SOC on the soil surface is that the disturbance of forests by anthropogenic activities may result in significant losses of SOC.
Contents of SOC did not differ between soil types, since, for example, the maximum and minimum values were from Andosols at a depth of 30 cm (Table 3), and at the depth from the profile with the least depth (72 cm; data not shown). Other factors that may have an influence on the variation of SOC at a landscape level are the topographic location, slope steepness, and floristic composition (Birkeland, 1984; Amundson, 2001; Johnson et al., 2011).
Water retention
The percentages of water retention at FC, WP, and AD had a broad variation among soil layers of the profiles, with the following intervals: 36 to 94 %, 14 to 52 %, and 6 to 45 %, respectively (Table 2). The percentage of humidity at FC correlated positively with the percentage of limes (R=0.5; p=0.001) and the concentration of SOC (R=0.74; p≤0.0001), and negatively with bulk density (R=-0.77; p≤0.0001). The multiple regression model showed that two variables, bulk density and the concentration of SOC accounted for 69 % of the variation in the magnitude of FC in the soil (R2=0.69; Table 5). The percentage of humidity in the WP had a positive correlation with the percentage of limes (R=0.38; p=0.02) and the concentration of SOC (R=0.53; p=0.001), and a negative correlation with the bulk density (R=-0.54; p≤0.001) and the percentage of sands (R=-0.33; p=0.045). According to the multiple regression model, the bulk density and the percentage of sands explained 49 % variation of the WP (Table 5). The proportion of AD had a positive correlation with the concentration of SOC (R=0.57; p≤0.0001) and the percentage of limes (R=0.36; p=0.027), and a negative one with bulk density (R=-0.60; p≤0.001) and percentage of clay (R=-0.47; p=0.003). The regression model showed that the concentration of SOC and the percentage of clay explained 44 % of variation in the magnitude of the AD (Table 5).
Table 5 Stepwise multiple regression analysis to evaluate the effect of the bulk density (BD) and the concentrations of organic carbon (SOC), sands (S), limes (L), and clay (R) on the retention of humidity at 1500 kPa (WP), the retention of humidity at 30 kPa (FC) and available water (WA) in soils of a cloud forest. The data set comes from eight profiles with their horizons (n=37). The models only include the variables that had a significant effect on the magnitude of the dependent variable.

Humidity retention in the soil is affected by texture, bulk density, and the concentration of organic matter (Gupta and Larson 1979; Rawls et al., 1982; Rawls et al., 2003). However, it is difficult to interpret the independent effect of such soil properties since these interact with each other. Thus, our results show a positive correlation between density and percentage of clay (R=0.62; p≤0.0001) and a negative correlation between bulk density and concentration of SOC (R=0.67; p≤0.0001). Saxton and Rawls (2006) suggest that texture has a prevailing role on water retention at high tensions (e.g. 1500 kPa), due to the important influence the size of soil particles has on water adsorption. These authors point out that the role of organic matter on water retention is more relevant at lower tensions (e.g. 33 kPa), since its influence on humidity takes place mainly (though not exclusively) through its effect on soil aggregation and water conductivity. Our results coincide with these appreciations. For example, the proportion of sands and bulk density stood out for their negative influence on humidity at WP (1500 kPa), whereas SOC stood out for its positive influence on the magnitude at AW (Table 5). The FC (30 kPa), which includes the humidity retained at high (WP) and low tensions (WA), was negatively influenced by the bulk density, and positively by the SOC (Table 5).
Water contents at FC and WP estimated at the total depth of the profiles varied from 249 to 510 L m-2 and from 98 to 284 L m-2, respectively (Table 3). These values are in the low part of the interval reported for Andosols. Thus, in forests of Cofre de Perote, Mexico, a water content at FC was estimated between 322 and 980 L m-2 in soil profiles with depths of 70 and 226 cm, respectively (Gamboa and Galicia, 2012); meanwhile, soils in forests of the Chichinautzin mountains showed water contents at FC among 612 and 1357 L m-2 at depths between 93 and 293 cm, respectively (Peña-Ramírez et al., 2009). These differences may be due to a higher bulk density in our soils than in those of other studies, which report densities below 0.9 g cm-3 in all the profiles studied, as well as to differences in the depths of the profiles analyzed, since those studies analyzed deeper soil profiles than in our study.
The water absorption area, according to the average distribution of roots in the profiles, ranged between 0 and 60 cm in depth. In this area, available water was estimated in 107 to 167 L m-2 (Table 3). It is worth mentioning that there were no differences in the availability of water between soils, since, as in the variation of the SOC, the highest and lowest AW values were found in Andosols. This lack of relation between AW and soil type may be related to the fact that the main factors that influenced the AW were the concentration of carbon and bulk density, which may be influenced more by the characteristics of the flora (e.g. root density) than by the type of soil (Jobbágy and Jackson, 2000; Schoenholtz et al., 2000).
Litter
The litter mass had a wide variation interval between sites, between 10.0 ± 2.4 and 19.7 ± 3.2 Mg ha-1, with an average of 15 ± 1.3 Mg ha-1. Assuming a concentration of carbon in organic matter of 50 % (IPCC, 2003), these values equal a stock of organic carbon of 5.0 ± 1.2 to 9.8 ± 1.6 Mg C ha-1, with an average of 7.5 ± 0.6 Mg C ha-1. The average of mass is greater than the values reported by Tanner (1980) (8.1 and 11.7 Mg ha-1) and McDonald et al. (2000) (4.3 Mg ha-1) for different CFs in Jamaica, and by Negrete-Yankelevich et al. (2007) (2.0-4.2 Mg ha-1) for a CF in Mexico. The highest accumulation of litter in our study’s CF suggests that here the rate of decomposition of organic matter is slow. In CFs, the low decomposition rate of the litter has been related to low concentrations of nutrients in the organic matter, low ambient temperatures, and with the generation of anaerobic conditions as a consequence of the high soil moisture (Benner et al., 2010; Giambelluca and Gerold, 2011). Considering the litter and the mineral soil, the stockof carbon in the soil fluctuated between 99 and 157 Mg ha-1, with an average of 126 ± 7 Mg ha-1.
Conclusions
This study produced information on soil from a scarcely studied cloud forest in Michoacán. The main soil groups were identified at a second level in little detail, and data is reported on organic carbon contents and retention of water in soils. The results may be used in carbon inventories for the state and countrywide to evaluate the role played by the terrestrial ecosystems in the carbon cycle, as well as to analyze the function of such ecosystems in the water cycle.
Carbon concentration decreased with soil depth, and 55 % of the total of carbon was concentrated in the 30 cm below the soil surface. The average content of carbon in the soil was among the lowest values reported for cloud forests in Mexico, and in the mid-section of the interval reported for Andosols in the Trans-Mexican Volcanic System.
Water retention at field capacity was negatively affected by bulk density and positively, by the concentration of carbon. The permanent wilting point was negatively affected by the bulk density and by the proportion of sands. The available water was positively affected by the concentration of carbon, highlighting the influence of organic matter in the availability of water for plants. Water retention showed low values in comparison to those reported for Andosols in the Trans-Mexican Volcanic System, presumably due to the higher bulk density of the soils in this study.
Given that the highest proportion of organic carbon is concentrated in the first 30 cm of the soil, this stock and water retention may be highly sensitive to natural and anthropogenic disturbances in the studied forest.
Literatura Citada
Álvarez-Arteaga, G., N. E. García, P. Krasilnikov, y F. García-Oliva. 2013. Almacenes de carbono en bosques montanos de niebla de la sierra norte de Oaxaca, México. Agrociencia 47: 171-180. [ Links ]
Amundson, R. 2001. The carbon budget in soils. Annu. Rev. Earth Planet. Sci. 29: 535-562. [ Links ]
Batjes, N. H. 1996. Development of a world data set of soil water retention properties using pedotransfer rules. Geoderma 71:31-52. [ Links ]
Benner, J., P. M. Vitousek, and R. Ostertag. 2010. Nutrient cycling and nutrient limitation in tropical montane cloud forests. In: Bruijnzeel L. A., F. N. Scatena, and L. S. Hamilton (eds). Tropical Montane Cloud Forests: Science for Conservation and Management. Cambridge University Press, London. pp: 90-100. [ Links ]
Binkley, D. and Fisher, R. 2013. Ecology and management of forest soils. John Wiley & Sons. Fourth Edition. 347 p. [ Links ]
Birkeland, W. P. 1984. Soils and Geomorphology. Oxford University Press. 372 p. [ Links ]
Bravo-Espinosa, M., M. Mendoza, T. Carlón-Allende, L. Medina, J. T. Sáenz, and R. Páez. 2012. Effects of converting forest to avocado orchards on loss ground cover and topsoil properties in the Trans-Mexican Volcanic System. Land Degrad. Dev. 25:452-457. [ Links ]
Bruijnzeel, L. A. and J. Proctor. 1995. Hydrology and biogeochemistry of tropical montane cloud forests: what do we really know? In: Hamilton, L. S., J. O. Juvik, and F. N. Scatena (eds). Tropical Montane Cloud Forests. Ecological Studies 110, Springer, New York. pp: 38-78. [ Links ]
Campos-Cascaredo, A., K. Oleschko, L. Cruz-Huerta, J. D. Etchevers, and C. Hidalgo. 2001. Estimation of allophane and its relationship with other chemical parameters in mountain andisols of the Volcano Cofre de Perote. Terra 19: 105-116. [ Links ]
Chapman, H. D. 1965. Cation-exchange Capacity. In: Black, C. A. (ed.). Method of Soil Analysis. Part 2. Chemical and Microbiological Properties. American Society of AgronomySoil Science Society of America, Inc. Madison, USA. pp: 891-900. [ Links ]
CONABIO (Comisión Nacional Para el Conocimiento y Uso de la Biodiversidad). 2010. El Bosque Mesófilo de Montaña en México: Amenazas y Oportunidades para su Conservación y Manejo Sostenible. CONABIO, México, D.F. 196 p. [ Links ]
Cotler, H. 2003. Características y manejo de suelos en ecosistemas templados de montaña. In: Sánchez, Ó., E. Vega, E. Peters, y O. Monroy-Vilchis (eds). Conservación de los Ecosistemas Templados de Montaña en México. INE, México, D.F. pp: 153-162. [ Links ]
De Jong, B. H., M. A. Cairns, P. K. Haggerty, N. Ramirez-Marcial, S. Ochoa-Gaona, J. Mendoza-Vega, and I. March-Mifsut. 1999. Land-use change and carbon flux between 1970s and 1990s in central highlands of Chiapas, Mexico. Environ. Manage. 23: 373-385. [ Links ]
Don, A., J. Schumacher, and A. Freibauer. 2011. Impact of tropical land‐use change on soil organic carbon stocks-a meta‐ analysis. Glob. Chang. Biol. 17: 1658-1670. [ Links ]
Elliott, E. T., J. W. Heil, E. F. Kelly, and H. C. Monger. 1999. Soil structural and other physical properties. In: Robertson, G., D. Coleman, C. Bledsoe, and P. Sollins (eds). Standard Soil Methods for Long-term Ecological Research. Oxford University Press Inc., NY. Pp: 74-85. [ Links ]
FAO (Food and Agriculture Organization). 2006. Guidelines for soil description. FAO, Rome. Pp 5-6. [ Links ]
Gamboa, A. M. and L. Galicia. 2012. Land-use/cover change effects and carbon controls on volcanic soil profiles in highland temperate forests. Geoderma 170: 390-402. [ Links ]
García-Oliva, F., R. L. Sanford Jr, and E. Kelly. 1999. Effects of slash-and-burn management on soil aggregate organic C and N in a tropical deciduous forest. Geoderma 88: 1-12. [ Links ]
Garduño-Monroy, V. H., P. Corona-Chávez, I. Israde-Alcántara, L. Mennella, E. Arreygue, B. Bigioggero, y S. Chiesa. 1999. Carta Geológica de Michoacán 1:250,000. 111 p. [ Links ]
Gee, G. W. and J. W. Bauder. 1986. Particle-size analysis. In: Klute, A. Methods of Soil Analysis. Part 1. Physical and Mineralogical Methods. American Society of Agronomy-Soil Science Society of America, Inc. Madison, USA. pp 383-411. [ Links ]
Giambelluca, T. and G. Gerold. 2011. Hydrology and biogeochemistry of tropical montane cloud forests. In: Forest Hydrology and Biogeochemistry. Springer, Netherlands. Pp: 221-259. [ Links ]
Gómez-Tuena A., T. Orozco-Ezquivel, y L. Ferrari. 2005. Petrogénesis ígnea de la faja volcánica trans-mexicana. Bol. Soc. Geol. Mex. 3: 227-283. [ Links ]
González-Espinosa, M., J. A. Meave, F. G. Lorea-Hernández, G. Ibarra-Manríquez, and A. C. Newton. 2011. The red list of mexican cloud forest trees, fauna and flora international. Cambridge, UK. 120 p. [ Links ]
Guo, L. B. and R. M. Gifford. 2002. Soil carbon stocks and land use change: a meta analysis. Glob. Change. Biol. 8: 345-360. [ Links ]
Gupta, S. and W. Larson. 1979. Estimating soil water retention characteristics from particle size distribution, organic matter percent, and bulk density. Water Resour. Res. 15: 1633-1635. [ Links ]
Hamilton, L. S., J. O. Juvik, and F. N. Scatena. 1995. The Puerto Rico tropical cloud forest symposium: introduction and workshop synthesis. In: Hamilton, L. S., J. O. Juvik, and F. N. Scatena (eds). Tropical Montane Cloud Forests. Ecological Studies 110. Springer Verlag, New York. pp: 1-18. [ Links ]
Hill, T., and P. Lewicki. 2007. STATISTICS: Methods and Applications. StatSoft, Tulsa, OK. [ Links ]
Huffman E. W. D. 1977. Performance of a new carbon dioxide coulometer. Microchem. J. 22: 567-573. [ Links ]
IMTA (Instituto Mexicano de Tecnología del Agua). 2013. Extractor Rápido de Información Climatológica III, v. 3.2. Información climatológica disponible en formato electrónico. Jiutepec, Morelos, México. [ Links ]
INEGI (Instituto Nacional de Estadística y Geografía). 2007. Conjunto de datos vectorial edafológico escala 1:250 000 Serie II. INEGI, Aguascalientes, México. [ Links ]
IPCC (Intergovernmental Panel on Climate Change). 2003. Good Practice Guidance for Land Use, Land-use change, and forestry. IPCC National Greenhouse Gas Inventories Programme. IGES, Japan. 300 p. [ Links ]
ISO 11265. 1994. Soil quality Determination of the specific electrical conductivity. International Organization for Standardization. 3 p. [ Links ]
ISRIC (International Soil Reference and Information Centre) 2002. Procedures for soil analysis. Technical paper 9. FAO, Italia. pp: 14-17. [ Links ]
IUSS Working Group WRB. 2014. World Reference Base for Soil Resources 2014. International Soil Classification System for Naming Soils and Creating Legends for Soil Maps. World Soil Resources Reports No. 106. FAO, Rome. 181 p. [ Links ]
Jobbágy, E. G., and R. B. Jackson. 2000. The vertical distribution of soil organic carbon and its relation to climate and vegetation. Ecol. Appl. 10: 423-436. [ Links ]
Johnson, K. D., F. N. Scatena, and Silver W. L. 2011. A typical soil carbon distribution across a tropical steepland forest catena. Catena 87: 391-397. [ Links ]
Kitayarna, K. 1995. Biophysical conditions of the montane cloud forests of Mount Kinabalu, Sabah, Malaysia. In: Hamilton L. S., J. O. Juvic, and F. N. Scatena (eds). Tropical Montane Cloud Forests. Springer-Verlag, NY. USA. pp: 183-197. [ Links ]
Klute, A. 1986. Water retention: laboratory methods. In: Klute, A. Methods of Soil Analysis. Part 1. Physical and Mineralogical Methods. American Society of Agronomy-Soil Science Society of America, Inc. Madison, USA. pp: 635-662. [ Links ]
Lützow, M. V., I. Kõgel-Knabner, K. Ekschmitt, E. Matzner, G. Guggenberger, B. Marschner, and H. Flessa. 2006. Stabilization of organic matter in temperate soils: mechanisms and their relevance under different soil conditions - a review. Eur. J. Soil Sci. 57: 426-445. [ Links ]
Malone, B.P., A. B. McBratney, V. Minasny, and G. M. Laslett. 2009. Mapping continuous depth functions of soil carbon storage and available water capacity. Geoderma 154: 138-152. [ Links ]
Martínez, M. L., O. Pérez-Maqueo, G. Vázquez, G. Castillo-Campos, J. García-Franco, K. Mehltreter, M. Equihua, and R. Landgrave. 2009. Effects of land use change on biodiversity and ecosystem services in tropical montane cloud forests of Mexico. For. Ecol. Manag. 258: 1856-1863. [ Links ]
McDonald, M. A. and J. R. Healey. 2000. Nutrient cycling in secondary forests in the Blue Mountains of Jamaica. For. Ecol. Manage. 139: 257-278. [ Links ]
Mendoza, M. E., E. López-Granados, D. Geneletti, D. R. Pérez-Salicrup, and V. Salinas. 2011. Analysing land cover and land use change processes at watershed level: a multitemporal study in the lake Cuitzeo Watershed, Mexico (19752003). Appl. Geog. 31: 237-250. [ Links ]
Negrete-Yankelevich, S., C. Fragoso, A. C. Newton, and O. W. Heal. 2007. Successional changes in soil, litter and macroinvertebrate parameters following selective logging in a Mexican Cloud Forest. Appl. Soil Ecol. 35: 340-355. [ Links ]
Osman, K. T. 2013. Soils: Principles, Properties and Management. Springer, London UK. 306 p. [ Links ]
Palm, C., P. Sanchez, S. Ahamed, and A. Awiti. 2007. Soils: A contemporary perspective. Annu. Rev. Environ. Resour. 32: 99-129. [ Links ]
Parfitt, R. L. and B. Clayden. 1991. Andisols: The development of a new order in Soil Taxonomy. Geoderma 49: 181-198. [ Links ]
Peña-Ramírez, V. M., L. Vázquez-Selem, and C. Siebe. 2009. Soil organic carbon stocks and forest productivity in volcanic ash soils of different age (1835-30,500 years BP) in Mexico. Geoderma 149: 224-234. [ Links ]
Pérez-Ramírez, S., M. I. Ramírez, P. F. Jaramillo-López, y F. Bautista. 2013. Contenido de carbono orgánico en el suelo bajo diferentes condiciones forestales: reserva de la biosfera mariposa monarca. RCHSCFA 19: 158-173. [ Links ]
Prentice, I. C., G. D. Farquhar, M. J. Fasham, M. L. Goulden, M. Heimann, V. J. Jaramillo, H. S. Kheshgi, C. L. Quéré, R. J. Scholes, and D. W. Wallace. 2001. The carbon cycle and atmospheric carbon dioxide. In: Houghton, J. T., Y. Ding, D. J. Griggs, M. Noguer, P. J. van der Linden, X. Dai, K. Maskell, and C. A. Johnson (eds). Climate Change 2001. The Scientific Basis. Contribution of Working Group I to the Third Assessment Report of the IPCC. Cambridge University Press. Cambridge, UK. pp 183-37. [ Links ]
Rasse, D. P., C. Rumpel, and M. F. Dignac. 2005. Is soil carbon mostly root carbon? Mechanisms for a specific stabilization. Plant Soil 269: 341-356. [ Links ]
Rawls, W. J., Y. A. Pachepsky, J. C. Ritchie, T. M. Sobecki, and H. Bloodworth. 2003. Effect of soil organic carbon on soil water retention. Geoderma 116(1), 61-76. [ Links ]
Rawls, W. J., D. L. Brakensiek, and K. E. Saxton. 1982. Estimation of soil water properties. T. Asae 25: 1316-1320. [ Links ]
Rumpel, C. and I. Kögel-Knabner. 2011. Deep soil organic matter a key but poorly understood component of terrestrial C cycle. Plant Soil 338: 143-158. [ Links ]
Rzedowski, J. 1996. Análisis preliminar de la flora vascular de los bosques mesófilos de montaña de México. Acta Bot. Mex. 35: 25-44. [ Links ]
Santana, H. G., M. E. Mendoza, M. V. Salinas, D. R. Perez-Salicrup, R. Y. Martínez, and M. I. Aburto. 2014. Análisis preliminar de la diversidad y estructura arbórea-arbustiva del bosque mesófilo en el sistema volcánico transversal de Michoacán, México. Rev. Mex. Biod. 85: 1104-1116. [ Links ]
Saxton, K. E. and W. J. Rawls. 2006. Soil water characteristic estimates by texture and organic matter for hydrologic solutions. Soil Sci. Soc. Am. J. 70: 1569-1578. [ Links ]
Schoenholtz, S. H., H. Van Miegroet, and J. A. Burger. 2000. A review of chemical and physical properties as indicators of forest soil quality: challenges and opportunities. For. Ecol. Manage. 138: 335-356. [ Links ]
Siebe, C., R. Jahn, and K. Stahr. 2006. Manual para la Descripción y Evaluación Ecológica de Suelos en el Campo. Sociedad Mexicana de la Ciencia del Suelo. México, Universidad Autónoma Chapingo, México. 2a edición. 57 p. [ Links ]
Shoji, S., M. Nanzio, and R. A. Dahlgren. 1993. Volcanic Ash Soils. Genesis, Properties, and Utilization. Dev. Soil Sci. 21. Elsevier, Amsterdam, The Netherlands. 287 p. [ Links ]
Shoji, S., M. Nanzio, R. A. Dahlgren, and P. Quantin. 1996. Evaluation and proposed revisions of criteria for Andosols in the World Reference Base for Soil Resources. Soil Sci. 161:605-615. [ Links ]
Shoji, S., T. Ito, M. Saigusa, and I. Yamada. 1985. Properties of nonallophanic Andisols from Japan. Soil Sci. 148: 264-277. [ Links ]
Tanner, E. V. J. 1980. Studies on the biomass and productivity in a series of montane rain forests in Jamaica. J. Ecol. 68:573-588. [ Links ]
Toledo-Aceves, T., J. A. Meave, M. González-Espinosa, and N. Ramírez-Marcial. 2011. Tropical montane cloud forests: current threats and opportunities for their conservation and sustainable management in Mexico. J. Environ. Manage. 92:974-981. [ Links ]
Villaseñor, J. L. 2010. El bosque húmedo de montaña en México y sus plantas vasculares: catálogo florístico-taxonómico. Comisión Nacional para el Conocimiento y Uso de la Biodiversidad/Universidad Nacional Autónoma de México, México, DF. 42 p. [ Links ]
Zar, J. H. 1999. Biostatistical Analysis. Englewood Cliffs, NJ, Prentice-Hall. 620 p. [ Links ]
Received: March 2015; Accepted: October 2015