Introduction
Fusarium oxysporum (Fol) causes plant wilting disease characterized by severe vascular damage and root rot, attacking a wide variety of crops of high human consumption, in tomato (Solanum lycopersicum L.) can induce serious yield losses (de Lamo & Takken, 2020). This pathogen increases reactive oxygen species (ROS) causing the antioxidant system to be unable to reduce such accumulation, which causes mutation of nucleic acids, protein oxidation and cellular damage (Sousa et al., 2015). These events cause a reduction in the availability, absorption, distribution and use of nutrients in plants (Romero et al., 2011), as well as photosynthesis decrease (Mo et al., 2017), thus reducing the growth and productivity of tomato plants as well as the quality of the fruits (Leyva-Mir et al., 2013). To mitigate this damage chemical control is the main management measure of this phytopathogenic disease (Solarte et al., 2012). Unfortunately, the indiscriminate application of chemicals has originated the development of resistant fungal populations (Ramírez et al., 2020). Thus, the implementation of alternatives to decrease the incidence and severity of Fol wilt of the disease induced by F. oxysporum to avoid crop losses is of great importance.
One of the most abundant elements in the lithosphere is silicon (Si). In soil solution, Si is present as silicic acid (H4SiO4, pKa = 9.25), which pH lower than 9, is the only form absorbed by the root (López-Pérez et al., 2018; Paye et al., 2018; van den Berg et al., 2021). Uptake this element into crop is accomplished by rejective, passive and active processes, is transported via the xylem through the transpiration current with the help of transporters, however, it is species-specific and complex (Frick et al., 2020; H. Sun et al., 2020). Supplement of this mineral is important because optimizes plants growth, productivity and quality parameters, in addition to biotic stress resistance (Dawa et al., 2020; Malhotra et al., 2016). The function of silicic acid against diseases induced by fungi, bacteria, and virus is given by induction of multiple mechanisms (Hoffmann et al., 2020). Physical barrier is occurs through silicic acid deposition and polymerization in cell structures (Islam et al., 2020), biochemical defense is constitutes by phenylalanine ammonia lyase (PAL); who has an important role in the synthesis of lignin and phenolic compounds, and antioxidant enzymes production as peroxidase (POD), ascorbate peroxidase (APX), catalase (CAT), superoxide dismutase (SOD) (Gulzar et al., 2021), in addition to non-enzymatic antioxidants, as phenolic compounds, flavonoids, among others (Jafari et al., 2015), as well as, phytohormones, and expression of pathogenesis-induced plant defense-related genes, as salicylic, jasmonic, abscisic acid, among others (Bhatt & Sharma, 2018). In different research studies, the effect of Si supplementation in agricultural crops has been reported. The calcium silicate application controlled the disease caused by Fusarium solani in Piper nigrum (DꞌAddazio et al., 2020). The same pathogen also was controlled with the supply of silicon dioxide nanoparticles to Daucus carota (Siddiqui et al., 2020). Ouzounidou et al. (2016) found that potassium silicate supplementation caused increased antioxidant content in Cucumis sativus. Similarly, Madany et al. (2020) found positive effect of silicon nanoparticles on Lycopersicon esculentum by enhanced enzymatic and non-enzymatic antioxidant activity.
Considering this information, the study aimed to evaluate the application of silicic acid, on antioxidant capacity, salicylic and jasmonic acid and defense genes expression in tomato crop inoculated with F. oxysporum.
Methods
Crop development
The development of the experiment was carried out in a greenhouse multi tunnel type, with polyethylene cover. As a biological material, tomato seedlings (Solanum lycopersicum L.) cv. “Rio Grande” was used, which were transplanted in 10 L black polyethylene bags, which contained substrate of peat moss and perlite in 1:1 proportion. For the nutritional management of the tomato crop a Steiner fertilizer solution was applied (Steiner, 1961). The tomato crop was developed during 119 days after transplanting (dat).
Silicic acid application
The application of silicic acid (ASi, 99.9%, Sigma-Aldrich, St. Louis, MO, USA) consisted of foliar spraying of this compound on the abaxial and adaxial sides of the whole plant; it was applied to following treatments Asi and Asi+Fol (18 plants each). A total of 10 applications of ASi were carried out, two applications were made prior to the inoculation of the pathogens (7 and 14 dat), and eight subsequent applications with intervals of one week. The concentration of silicic acid used was 110 g ha-1 per application.
Inoculation of Fusarium oxysporum
Fusarium oxysporum strain (Fol) was used as inoculum; strain from the Laboratory of Molecular Parasitology, Universidad Autónoma Agraria Antonio Narro (Limon-Corona et al., 2022). To carry out the inoculation of F. oxysporum (Fol), it was first reactivated, with the objective of increasing the viability of the latent inoculum (Coninck et al., 2020), in potato dextrose agar (PDA), and incubated for 15 days at 27 °C (Benhamou & Bélanger, 1998). For the increase of the pathogen conidia a semiliquid medium was used mixing potato, dextrose, PDA, streptomycin and tomato seedling; in this medium two mycelial discs (Ɵ = 6 mm) were sowing and it was incubated at 28 °C in an orbital shaker at 168 × g 125 rpm by 15 days. At 21 days after transplantation, the tomato plants of the Fol and ASi+Fol treatments were inoculated at a concentration of 1×106 spores mL-1 resuspended in distilled water, applying 60 mL of the conidia solution on perforations in the substrate (10 cm deep and 3 cm away from the stem) (Benhamou & Bélanger, 1998). Fol inoculum was not applied to the plants of treatments ASi and T0.
Description of treatments
Four treatments were evaluated ASi+Fol, ASi, Fol and T0. ASi+Fol treatment consisted of the inoculation of Fol plus the foliar application of silicic acid. ASi treatment consisted of the foliar application of silicic acid. Fol treatment consisted of the inoculation of Fol without Asi application. Finally, T0 (absolute control) treatment was not inoculated with Fol and Asi was not sprayed. A total of 18 plants were used per treatment.
Incidence and severity of Fusarium oxysporum wilt disease
Incidence and severity of Fol wilt parameters were recorded at 43, 54, 58, 61, 66, 70, 72, 74, 76, 78, 82 y 85 dat. Disease incidence on tomato plants was determined visually by the presence or absence of symptomatic plants. Therefore, zero represented asymptomatic plants and 1 represented symptomatic plants. All data were expressed in percentage. The severity of Fol wilt disease was determined following the visual scale of Diener & Ausubel, (2005) and González-García et al. (2023); which is described as follows: 0: plants indistinguishable from mock; 1 = wilting of basal leaves (from the fifth true leaf); 2 chlorosis of basal leaves (from the fifth true leaf) and wilting of young leaves; 3 = wilting of most of the leaves, diffuse desiccation and yellowing; 4 = dead plant. Disease severity is calculated as a percentage using the following equation:
Biochemical variables
The biomolecules: proteins, catalase, ascorbate peroxidase, superoxide dismutase, glutathione peroxidase, phenylalanine ammonium lyase, reduced glutathione, ABTS, and DPPH antioxidant capacity were extracted of leaf tissue. For this purpose, after 73 days of transplantation, random plants were selected, and the third fully expanded young leaf was taken for biochemical analysis. The tissue was lyophilized, macerated in a mortar and stored (-80 °C) for subsequent analyses. For the enzymatic and non-enzymatic determination, 200 mg of lyophilized and macerated leaves of each treatment and 20 mg of polyvinylpyrrolidone were weighed. After this, 1.5 mL of phosphate buffer with a pH of 7-7.2 (0.1 M) were added, and the mixture was then subjected to micro-centrifugation at 16,128 × g 12,000 rpm for 10 min at 4 °C. The supernatant was filtered with a 0.45 μm pore size nylon membrane (Ramos et al., 2010).
Total protein quantification was determined by the method of Bradford, (1976), the results were expressed as mg g-1 dry weight (DW). Catalase (EC 1.11.1.6) activity was quantified by measuring 2 reaction times according to Dhindsa et al. (1981), the results were expressed as U g-1 TP (U per gram of total proteins) of DW, where U is equal to the mmol equivalent of H2O2 consumed per milliliter per minute. Ascorbate peroxidase (EC 1.11.1.1) was determined according to Nakano & Asada, (1981), the results were expressed as U g-1 TP of DW, where U is equal to the μmol equivalent of oxidized ascorbate per milliliter per minute. Superoxide dismutase (EC 1.15.1.1) was determined was carried out using the Cayman kit (SOD Assay Kit 706002®, Cayman Chemical, Ann Arbor, Michigan, USA), the results were expressed as U mL−1 of DW, where U is defined as the amount of enzyme needed to exhibit 50% dismutation of the superoxide radical. Glutathione peroxidase (EC 1.11.1.9) was conducted according to Flohé & Günzler, (1984) technique, using H2O2 as a substrate, the results were expressed as U g-1 TP of DW, where U is equal to the mmol equivalent of reduced glutathione per milliliter per minute. Phenylalanine ammonia lyase (EC 4.3.1.5) was determined according to Sykłowska-Baranek et al. (2012), the results were expressed as U 100 g-1 TP of DW, where U is equal to production of 1 µmol cinnamic acid per milliliter per minute. Reduced glutathione (GSH) was performed colorimetrically by reaction with DTNB (Xue et al., 2001), the results were expressed as µmol g-1 of DW.
ABTS (2,2 ’-azino-bis(3-Ethylbenzotiazoline-6-Sulphonic Acid)) was performed according to the methodology of Re et al. (1999), the results were expressed as equivalents vitamin C µg g−1 of DW. DPPH (2,2-diphenyl-1-Picrylhydrazyl) was performed using the method of Brand-Williams et al. (1995), the results were expressed as equivalents vitamin C µg g−1 of DW.
Phenols were determined according to the methodology of Yu & Dahlgren, (2000), the results were expressed as equivalents gallic acid mg g−1 of DW. Flavonoids was performed with the method of Arvouet-Grand et al. (1994), the results were expressed as equivalents of quercetin mg g−1 of DW. Vitamin C was determined by the titration method with 2,6 dichlorophenolindophenol (Padayatt et al., 2001), the results were expressed as mg 100 g−1 fresh weight.
The content of lycopene, β-carotene, and a y b chlorophylls were determined according to Nagata & Yamashita (1992). Total chlorophyll was the sum of Chl a and Chl b. All data are expressed as mg 100 g−1 of DW.
Endogenous phytohormones
To determine salicylic acid (SA), 1 mL of the extraction solution (89% water, 10% methanol and 1% acetic acid) was added to 50 mg of the foliar tissue. Subsequently, were centrifuged at 16,000 × g (10 minutes). The supernatant was extracted and filtered (0.45 μm filter) and degassed by sonication. A liquid chromatograph (Agilent compact 1120) equipped with variable wavelength UV detector was used. The chromatographic conditions were for stationary phase: column C-18 polaris of 250 mm in length. The mobile phase has a gradient of 50% phase A (water 94.9%, acetonitrile 5%, and formic acid 0.1%) and 50% phase B (acetonitrile 94.9%, water 5%, and formic acid 0.1%), at a flow of 0.8 mL min-1 for a run time of 13 min. It was read at a wavelength of 250 nm (Forcat et al., 2008). The results were expressed as mg kg-1 of DW.
Jasmonic acid (JA) was determinate mixing 100 mg of leaf tissue and 900 μL of the extraction solution (95% methanol and 5% ethyl acetate), vortexed, sonicated (10 min), and centrifuged at 16,000 × g (10 min). The supernatant was filtered, the solvent was subsequently evaporated in dry oven at 50 °C, and the residues were re-suspended in 500 μL of mobile phase (60 % methanol, 39% water, and 1% acetic acid). Same equip was used to determine jasmonic acid (JA). The flow was 0.60 mL min-1 using a Hypersil ODS column of 25 cm × 4.6 mm × 5 μm, at a wavelength of 230 nm with an analysis time of 20 min (Kramell et al., 1995; Michelena et al., 2001). The results were expressed as mg kg-1 of DW.
Determination of gene expression by qPCR
The RNA was extracted using 100 mg fresh leaf tissue and TRIzol reagent (TRI Reagent®, MRC, TR 118), purified with chloroform, and precipitated with isopropanol, as described in Cui et al. (2004). RNA quantification was carried out in a UV-Vis spectrophotometer (Thermo Scientific Model G10S) at an absorbance of 260 and 280 nm, and RNA quality was analyzed by denaturing electrophoresis in agarose gel. 2 µg of total-RNA were used by synthesis of the cDNA was performed using a commercial kit (Promega, Madison, Wisconsin, USA). The primers correspond to an endogenous gene ACT (actin) and six study genes: PR1 (salicylic acid), JA (jasmonic acid), GAME1 (phytoalexins), PAL5-3 (phenylalanine ammonia lyase), which were designed in the software AMPLIFIX, OLIGOANALIZER and Primer-BLAST. For the ET (ethylene) and ABA (abscisic acid), the sequences cited by Anstead et al. (2010) and Nitsch et al. (2009), were considered, respectively (Table 1).
Tabla 1 Secuencia de cebadores de los genes analizados.Tabla 1. Secuencia de cebadores de los genes analizados.
Gene | (Forward primer 5’-3’) | (Reverse primer 5’-3’) | Tm (°C) Annealing |
---|---|---|---|
ACT | CCCAGGCACACAGGTGTTAT | CAGGAGCAACTCGAAGCTCA | 60 °C |
PR1 | AAGTAGTCTGGCGCAACTCA | GTCCGATCCAGTTGCCTACA | 60 °C |
JA | TGGTTCGTCGACTTCGTCAT | CTCGGCCTTGAGAGAGTTCA | 60 °C |
GAME1 | TCGTTGTTCCTGGGTTACCT | TCCGAAACTCGAACTTTCTCG | 58 °C |
PAL5-3 | GGAGGAGAATTTGAAGAATGCTGTG | TCCCTTTCCACCACTTGTAGC | 60 °C |
ET | TGTCCCAAGCCAGACTTGAT | TGCCATCTTGTTGAGCAATC | 60 °C |
ABA | CTTATTTGGCTATCGCTGAACC | CCTCCAACTTCAAACTCATTGC | 60 °C |
The primers were prepared at a concentration of 15 pmol mL-1. The quantification method used was a standard relative curve, therefore, for each quantification analysis a standard curve per gene was included using a 1:5 dilution.
qPCR reactions were analyzed in an Applied Biosystems StepOne™ Equipment version 2.3 by the standard relative curve method, measuring the fluorescence intensity of SYBR Green. The qPCR reaction for all genes was performed in a total volume of 20 μL. Regarding ACT gene, 10 µL of SYBR® Select Master Mix (Applied Biosystems, Foster City, California, USA) were added along with 0.10 μL of first forward (72 nM), 0.08 μL of first reverse (60 nM), 1 μL of cDNA and 8.82 μL of nuclease-free water. Regarding the PR1 gene, 10 μL of Master Mix were added along with 0.03 μL of first forward (20 nM), 0.05 μL of first reverse (40 nM), 1 μL of cDNA diluted at a ratio of 1:5, and 8.92 μL of nuclease-free water. Regarding the JA gene, 10 μL of Master Mix, 0.05 μL of first forward (40 nM), 0.08 μL of first reverse (60 nM), 1 μL of cDNA and 8.87 μL of nuclease-free water were added. ABA gene, 10 μL of Master Mix, 4 μL of first forward (3000 nM), 4 μL of first reverse (3000 nM), 1 μL of cDNA and 1 μL of nuclease-free water were added. As for ET, PAL5-3 and GAME1 genes, 10 μL of Master Mix, 0.13 μL of first forward (100 nM), 0.13 μL of first reverse (100 nM), 1 μL of cDNA (ET and PAL5-3) or 1 μL of cDNA diluted 1:5 (GAME1) and 8.74 μL of nuclease-free water were added. The qPCR was run with the following program in the thermal cycler: Hot Start, 10 min at 95 °C and PCR (40 cycles), 15 s at 95 °C and 1 min at temperature annealing.
Statistical analysis
The experiment was stablished in completely random design. To estimate the incidence and severity of Fol wilt the disease 18 replicates per treatment were considered; and for biochemical, secondary metabolites and gene expression five replicates were considered per treatment. An analysis of variance and Fisher´s Least Significant Difference test (p ≤ 0.05) were performed using the InfoStat software (v2019).
Results
Incidence and severity of Fol wilt disease
Statistical differences were found between treatments in incidence of Fol wilt in tomato plants, throughout the experiment, except for the first sampling (Figure 1A). There was no incidence of Fol wilt the disease in silicic acid (ASi) treatment and absolute control (T0). As for Fol treatments (Fol and ASi+Fol) a certain degree of incidence of Fol wilt was observed throughout the evaluation dates but increased as culture developed during time. Particularly, it was observed that ASi application decreased incidence of Fol wilt as ASi+Fol treatment had a lower incidence of Fol wilt than the Fol treatment form inoculation day to day 66 after transplanting. Best effect of ASi was obtained at 61 days after transplanting (dat), since incidence of Fol wilt was reduced by approximately 24%. After 70 dat, both infested treatments reached 100% incidence.
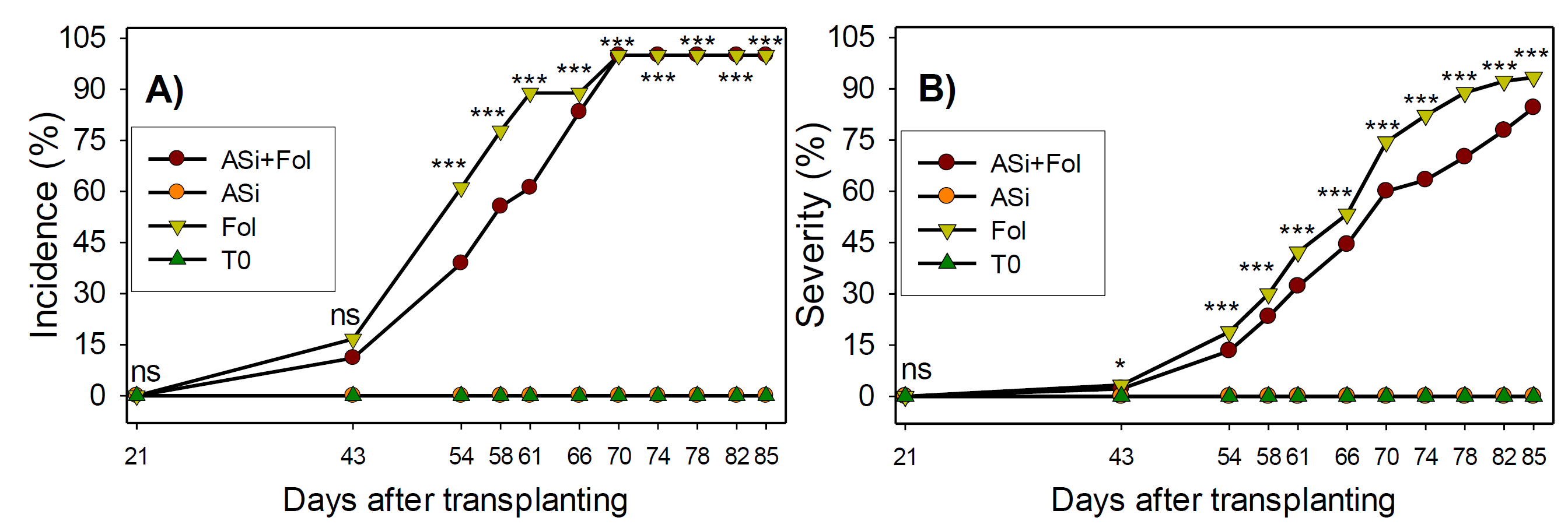
Figure 1 Incidence (A) and severity (B) of Fol wilt disease in tomato plants with or without ASi application. ns: not significant, *, ***: statistical differences at p < 0.05, < 0.0001 respectively. n= 18. ASi+Fol: silicic acid + F. oxysporum. ASi: silicic acid. Fol: F. oxysporum. T0: absolute control.
The first symptoms of the severity of phytopathogen were observed at 43 dat and increased progressively during culture development (Figure 1B). Throughout the experiment ASi+Fol treatment consistently decreased Fol severity. At 78 dat, the greatest decrease in severity was observed, with approximately 21% less incidence of wilt than Fol treatment, and 12% at 85 dat.
Biochemical Responses
Results showed statistical differences in total protein content, carotenoids, and chlorophylls in tomato leaves (Table 2). There was no difference in protein content between ASi and T0 treatments, whereas Fol and ASi+Fol treatments significantly decreased proteins by 33% and 31% respectively.
Table 2 Total protein, carotenoids, and chlorophyll content in leaves of tomato plants infested and not-infested with F. oxysporum and with or without ASi applicationsTabla 2. Contenido total de proteínas, carotenoides y clorofila en hojas de plantas de tomate infestadas y no infestadas con F. oxysporum, con o sin aplicaciones de ASi (ácido silícico).
Treatments | Proteins (mg g-1 DW) |
Lycopene (mg 100 g-1 DW) |
β-carotene (mg 100 g-1 DW) |
Chlorophyll a
(mg 100 g -1 DW) |
Chlorophyll b
(mg 100 g -1 DW) |
Total Chlorophyll (mg 100 g-1 DW) |
---|---|---|---|---|---|---|
ASi+Fol | 19.1b | 6.3ab | 41.9a | 180.8a | 67.8a | 248.6a |
ASi | 26.7a | 9.0a | 30.5ab | 206.4a | 71.3a | 277.7a |
Fol | 18.6b | 3.1b | 28.8ab | 92.1b | 40.3b | 132.4b |
T0 | 27.8a | 8.3a | 26.3b | 172.0a | 50.8ab | 222.7a |
CV (%) | 23.0 | 41.0 | 25.8 | 22.1 | 23.2 | 22.8 |
n= 5. Averages with same letter per column are statistically equal (LSD Fisher, p ≤ 0.05). ASi+Fol: silicic acid + F. oxysporum. ASi: silicic acid. Fol: F. oxysporum. T0: absolute control. DW: dry weight. CV: coefficient of variation (%).
n= 5. Medias con la misma letra por columna son estadísticamente iguales (LSD Fisher, p ≤ 0.05). ASi+Fol: ácido silícico + F. oxysporum. ASi: ácido silícico. Fol: F. oxysporum. T0: control absoluto. DW: peso seco. CV: coeficiente de variación (%).
Lycopene content in tomato leaves inoculated with Fol was 3.1 mg 100 g-1 DW; which corresponds to 51, 62 and 65% less than the content registered in the plants of the ASi+Fol, T0 and ASi treatments, respectively (Table 2). There was no statistically significant difference between the ASi+Fol, ASi and T0 treatments. In the case, β-carotene, there were no statistically significant differences between treatments, only the ASi+Fol treatment presented the highest content, with approximately 59% more than T0.
Regarding chlorophyll content (a, b and total), results were consistent. The total chlorophyll a content in the plants inoculated with Fol was 92.1, 40.3 and 132.4 mg 100 g-1 DW, respectively; this quantity was 49.1, 40.6 and 46.7%, respectively, lower than the content registered in the plants of the ASi+Fol treatments. There was no significant statistical difference between the ASi+Fol, ASi and T0 treatments (Table 2).
Significant differences between treatments were observed in vitamin C, flavonoids, phenols and antioxidant compounds, as well as in DPPH antioxidant capacity (Table 3). Vitamin C content no differences were found between the plants inoculated with Fol and the plants treated with ASi+Fol, however, the plants inoculated with Fol were 52.7 and 33.1% higher than the content presented in the plants treated with ASi and T0. The reduced glutathione was higher in plants inoculated with Fol, 69.5 and 6.6% higher than ASi+Fol and ASi plants. Phenol content did not present differences between the plants treated with Fol, ASi+Fol and ASi, only an increase of 32% stood out in the plants inoculated with Fol, with respect to T0. Regarding Flavonoid content, showed a reduction of 24.6, 27.8 and 30.4% in plants inoculated with Fol, compared to plants treated with ASi+Fol, T0 and ASi respectively. ABTS did not present differences between treatments, while antioxidant capacity, evaluated by DPPH increased 3.1% in Fol compared to ASi+Fol (Table 3).
Table 3 Non-enzymatic antioxidant compounds in leaves of tomato plants infested and not-infested with F. oxysporum and with or without ASi applications at 73 dat.Tabla 3. Compuestos antioxidantes no enzimáticos en hojas de plantas de tomate infestadas y no infestadas con F. oxysporum, con o sin aplicaciones de ASi (ácido silícico) a los 73 días después del trasplante (dat).
Treatments | Vitamin C (mg 100 g-1 FW) |
Reduced glutathione (µmol g-1 DW) |
Phenols (mg g-1 DW) |
Flavonoids (mg g-1 DW) |
ABTS (µg g-1 DW) |
DPPH (µg g-1 DW) |
---|---|---|---|---|---|---|
ASi+Fol | 123.2ab | 5.9b | 18.4ab | 28.9a | 7.7a | 52.4b |
ASi | 96.8b | 8.5ab | 17.2ab | 31.3a | 7.8a | 54.2a |
Fol | 147.8a | 10.0a | 20.4a | 21.8b | 8.9a | 54.0a |
T0 | 110.9b | 8.9ab | 15.2b | 30.2a | 9.5a | 53.7a |
CV (%) | 20.3 | 27.8 | 11.9 | 9.6 | 21.9 | 1.1 |
n= 5. Averages with the same letter per column are statistically equal (LSD Fisher, p ≤ 0.05). ASi+Fol: silicic acid + F. oxysporum. ASi: silicic acid. Fol: F. oxysporum. T0: absolute control. ABTS: 2,2'-azino-bis(3-ethylbenzthiazolin-6-sulfonic acid). DPPH: 2,2-diphenyl-1-picrylhydrazyl. CV: coefficient of variation (%). FW: fresh weight. DW: dry weight.
n= 5. Las medias con la misma letra por columna son estadísticamente iguales (LSD Fisher, p ≤ 0.05). ASi+Fol: ácido silícico + F. oxysporum. ASi: ácido silícico. Fol: F. oxysporum. T0: control absoluto. ABTS: ácido 2,2'-azino-bis(3-etilbenztiazolin-6-sulfónico). DPPH: 2,2-difenil-1-picrilhidrazil. CV: coeficiente de variación (%). FW: peso fresco. DW: peso seco.
Except for APX y SOD enzyme, there were statistical differences in PAL, CAT and GPX between treatments (Table 4). PAL no differences were found between the plants inoculated with Fol and the plants treated with ASI+Fol, however, the plants inoculated with Fol presented 3.2% higher activity than the plants treated with ASi and T0. The activity of the CAT enzyme was higher in the plants inoculated with Fol in 85.1 and 108.4 %, with respect to the plants of the ASi+Fol and T0 treatments. The enzymatic activity of GPX was similar in the plants of the Fol, ASi+Fol and ASi treatments, only an increase of 193% was observed in the plants inoculated with Fol, with respect to T0.
Table 4 Enzymatic activity in leaves of tomato plants infested and not-infested with F. oxysporum and with or without ASi application at 73 dat.Tabla 4. Actividad enzimática en hojas de plantas de tomate infestadas y no infestadas con F. oxysporum, con o sin aplicación de ASi (ácido silícico) a los 73 días después del trasplante (dat).
Treatments | PAL (U 100 g-1 TP) |
APX (U g-1 TP) |
CAT (U g-1 TP) |
GPX (U g-1 TP) |
SOD (U ml-1) |
---|---|---|---|---|---|
ASi+Fol | 4.0ab | 15.9a | 513.2b | 48.7a | 9.6b |
ASi | 3.2b | 9.6a | 623.9ab | 41.3a | 24.6a |
Fol | 5.5a | 14.3a | 949.7a | 58.5a | 16.1ab |
T0 | 3.2b | 16.5a | 455.7b | 19.6b | 25.3a |
CV (%) | 22.3 | 56.7 | 37.1 | 26.4 | 46.2 |
n=5. Averages with same letter per column are statistically equal (LSD Fisher, p ≤ 0.05). ASi+Fol: silicic acid + F. oxysporum. ASi: silicic acid. Fol: F. oxysporum. T0: absolute control. PAL: phenylalanine ammonia lyase. APX: ascorbate peroxidase. CAT: catalase. GPX: glutathione peroxidase. SOD: superoxide dismutase. TP: total protein. CV: coefficient of variation (%).
n=5. Las medias con la misma letra por columna son estadísticamente iguales (LSD Fisher, p ≤ 0.05). ASi+Fol: ácido silícico + F. oxysporum. ASi: ácido silícico. Fol: F. oxysporum. T0: control absoluto. PAL: fenilalanina amonio liasa. APX: ascorbato peroxidasa. CAT: catalasa. GPX: glutatión peroxidasa. SOD: superóxido dismutasa. TP: proteína total. CV: coeficiente de variación (%).
Endogenous phytohormones
Salicylic acid content in tomato leaves was modified by treatments in two out of three samplings, but no statistical differences were obtained between treatments at 31 dat (Table 5). At 73 dd, when the plants appeared an advanced severity (Figure 1B), the plants of the Fol, ASi+Fol and ASi treatments, did not emerge statistically significant directions, only the plants inoculated with Fol presented 247.5% higher concentration than the plants T0.
Table 5 Content of secondary metabolites related to defense systems in leaves of tomato plants infested and not-infested with F. oxysporum and with or without ASi applicationsTabla 5. Contenido de metabolitos secundarios relacionados con los sistemas de defensa en hojas de plantas de tomate infestadas y no infestadas con F. oxysporum, con o sin aplicaciones de ASi (ácido silícico).
Treatments | Salicylic acid (mg kg-1 DW) |
Jasmonic acid (mg kg-1 DW) |
||||
---|---|---|---|---|---|---|
15 dat | 31 dat* | 73 dat | 15 dat | 31 dat* | 73 dat | |
ASi+Fol | Nq | 35.1a | 171.6a | Nq | 5.7b | 72.1b |
ASi | 52.6a | 23.9a | 72.3ab | Nd | Nd | 39.1c |
Fol | Nq | 32.2a | 163.0a | Nq | 19.7a | 130.7a |
T0 | 21.9b | 43.9a | 46.9b | Nd | Nd | Nd |
CV (%) | 42.8 | 49.6 | 66.2 | - | 58.3 | 33.1 |
n=5. Averages with same letter per column are statistically equal (LSD Fisher, p ≤ 0.05). ASi+Fol: silicic acid + F. oxysporum. ASi: silicic acid. Fol: F. oxysporum. T0: absolute control. CV: coefficient of variation (%). DW: dry weight. *: 10 days after inoculation of Fol. Nq: not quantified. Nd: not detected (is present but conjugated, not separated).
n=5. Las medias con la misma letra por columna son estadísticamente iguales (LSD Fisher, p ≤ 0.05). ASi+Fol: ácido silícico + F. oxysporum. ASi: ácido silícico. Fol: F. oxysporum. T0: control absoluto. CV: coeficiente de variación (%). DW: peso seco. *: 10 días después de la inoculación con Fol. Nq: no cuantificado. Nd: no detectado (presente pero conjugado, no separado).
The content of JA could only be quantified at 31 and 73 dat, with statistical differences between treatments in both cases (Table 5). Systematically, an increase of JA was observed as culture developed and consequently the severity of the pathogen increased (Figure 1B). At 31 dat, when the disease had not yet proliferated, only JA was quantified in the ASi+Fol and Fol treatments, the latter being significantly higher (245.6%). At 73 dat, once the incidence and severity of Fol wilt increased (Figure 1), an increase JA was observed in all treatments, except for T0. Once again, Fol generated the highest concentration of JA, surpassing the ASi+Fol treatment by 81.3 %, and the ASi treatment by 234.3%.
Gene expression
PR1 and JA genes, which encode salicylic acid and jasmonic acid respectively, showed changes in their expression due to treatments (Figure 2). At 31 dat, only Fol treatment modified PR1 and JA gene expression, repressing them 0.7- and 0.34-fold change respectively in comparison to ASi+Fol. However, at 73 dat all treatments increased PR1 gene expression, however the combination of ASi+Fol generated the largest increase with 18.7-fold change, while ASi and T0 decreased it 2.2- and 2.5-fold change, respectively, in comparison to Fol (Figure 2A). This trend is consistent with what was observed in SA quantification, where T0 had the lowest amount of this metabolite and Fol treatments showed the highest amount (Table 5).
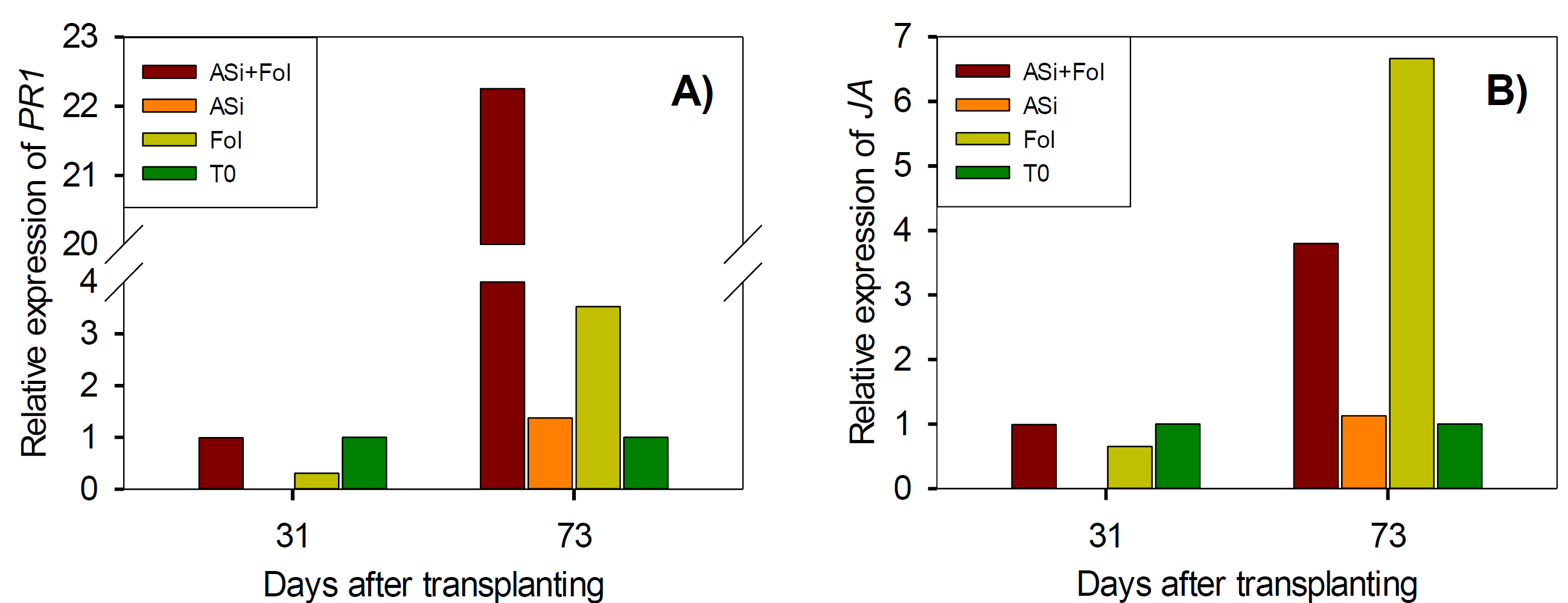
Figure 2 Relative expression of salicylic acid (PR1) (A) and jasmonic acid (JA) (B) genes in tomato leaves infested and not-infested with F. oxysporum and with or without ASi applications. ASi+Fol: silicic acid + F. oxysporum. ASi: silicic acid. Fol: F. oxysporum. T0: absolute control.
In the case of JA gene at 73 dat, in comparison at 31 dat, a similar trend was observed since all treatments increased its expression. However, ASi+Fol, ASi and Fol decreased 2.9-, 5.5- and 5.7-fold change, respectively, in comparison to Fol (Figure 2B). Results consistent with JA determination, since Fol generated the highest amount of this metabolite, followed by the combinations ASi+Fol and ASi (Table 5).
At 31 dat, application of the treatments practically showed no changes in GAME1 gene expression (Figure 3A). However, at 73 dat, the expression of this gene was repressed 0.1-fold change in ASi+Fol, and overexpressed ASi and T0 (1.3- and 0.7-fold change, respectively) compared to Fol.

Figure 3 Relative expression of phytoalexins (GAME1) (A) and phenylalanine ammonia lyase (PAL5-3) (B) genes in tomato leaves infested and not-infested with F. oxysporum and with or without ASi applications. ASi+Fol: silicic acid + F. oxysporum. ASi: silicic acid. Fol: F. oxysporum. T0: absolute control.
In the PAL5-3 gene, at 31 dat, a overexpression of this gene was observed by the ASi+Fol and T0 treatments, equivalent to 0.4- and 0.6-fold change, respectively, compared to Fol (Figure 3B). However, at 73 dat all treatments repression this gene, ASi+Fol was 0.2-fold change less, T0 was 1.8-fold change, and ASi generated the highest expression equivalent to 0.1-fold change more than Fol.
The expression of ET gene at 31 dat increased slightly by ASi+Fol (0.5-fold change), while T0 treatment decreased 0.1-fold change in comparison to Fol. However, at 73 dat all treatments overexpressed this gene: ASi+Fol, 5.5-fold change, ASi generated the highest expression with 7.1-fold change, except T0 (-1.7-fold change), greater than Fol (Figure 4A). In the case of ABA gene, at 31 dat Asi+Fol treatment generated a overexpression of 0.6-fold change in comparison to Fol, whereas ASi+Fol treatment increased the expression in 0.30-fold change, whereas T0 treatment increased the expression in 0.3-fold change (Figure 4B). At 73 dat, all treatments decreased ABA gene expression just like what was observed with the ET gene (T0) (Figure 4A). T0 treatment generated the lowest expression of the ABA gene with 4.5-fold change less, compared to Fol, followed by ASi treatment with 3.3-fold change. ASi+Fol treatment also decreased ABA gene expression but not in the same magnitude as T0 and ASi treatments, since it only increased 0.06-fold change in comparison to Fol (Figure 4B).

F. oxysporum. ASi: silicic acid. Fol: F. oxysporum. T0: absolute control.
Figure 4 Relative expression of ethylene (ET) (A) and abscisic acid (ABA) (B) genes in tomato leaves infested and not-infested with F. oxysporum and with or without ASi applications. T0: absolute control. ASi+Fol: silicic acid +
Discussion
Incidence and severity of Fol wilt disease
Cao et al. (2017) mentioned that when stress is prolonged, self-regulation in tomato is exceeded, and the positive effects of Si are attenuated, as observed here after 70 dat, where both infested treatments reached 100% incidence. Furthermore, without the use of fungicides, it is practically impossible to completely suppress the disease (Bakhat et al., 2018) which explains the results observed here (Figure 1A). Once the tissue is infected, the xylem vessels is obstructed by fungal structures or polysaccharides (Singh et al., 2017), there is also toxins secretion by this pathogen, this cause wilting and necrosis of the leaves, and dark brown vascular discoloration (Pirayesh et al., 2018). At the same time there is an excessive ROS production, causing oxidative stress, that generate DNA and metabolism damage, eventually culminating in the apoptosis (Aybeke, 2017). These symptoms produced together the high severity levels of Fol recorded in tomato plants under study (Figure 1B).
On the other hand, several authors have reported the different action mechanisms of silicic acid to mitigate the incidence and severity of Fol wilt disease, achieving a better plants development (Figure 1). In this work it was observed that the ASi and T0 treatments did not present symptoms of the disease, since were not inoculated with Fol, however the plants inoculated with Fol showed wilting of the leaves, likewise, in the plants treated with ASi+Fol but with a lower degree of incidence and severity (up to 24 and 21%, respectively) (Figure 1).
It was found that Si can activate the biochemical mechanisms of plants, such as increased antioxidant activity and phytohormones (Bakhat et al., 2018), in addition to pathogenicity related genes expression (Bhatt & Sharma, 2018). Once in the plant, Si can polymerize into amorphous silica and accumulate in the trichomes or cuticles of the leaves, forming a physical defense, which prevents the passage of pathogens (Dann & Le, 2017; Hoffmann et al., 2020). For example, silicic acid induces biochemical barriers, and once polymerized in amorphous silica, generates physical barriers (Bathoova et al., 2021; Dann & Le, 2017). In addition, its accumulation in cell walls, intracellular spaces and leaf trichomes generates firmer and more rigid structures (Carré-Missio et al., 2014; Ferreira et al., 2015). In the cytoplasm, it induces biochemical barriers such as metabolic pathways activation, JA (Bakhat et al., 2018; Ning et al., 2014), SOD and CAT activity (Cao et al., 2017) increase, flavonoid and PAL5-3 expression (Rahman et al., 2015). Silicic acid also participates in the overexpression or repression of pathogenicity related genes (Brunings et al., 2009).
Biochemical Responses
F. oxysporum produces toxins such as fusaric acid that causes oxidative stress induced by ROS, causing damage to the membrane, and reducing photosynthetic pigment content in tomato leaf tissue (Singh et al., 2017). In this work, proteins and lycopene clearly were affected by Fol, regardless of whether silicic acid is applied (Table 2). Opposite case, the chlorophylls were not reduced with the ASi+Fol treatment, it is possible that the application of ASi in Fol inoculated tomato plants protected against pigment degradation and enhance the photosynthetic capacity (S. Sun et al., 2022), because Chlorophyll content is related to higher photosynthetic capacity in plants under stress (González-García et al., 2023). It has been observed that Si applied on leaves to wheat under hydric stress increased chlorophyll concentration, improving light use efficiency (Maghsoudi et al., 2015). In Glycine max, Na2SiO3 application promoted Fe mobilization and maintenance in leaves, generating greater protection to chlorophyll degradation (Gonzalo et al., 2013).
Previously, it has been reported that pathogen infection in plants induces oxidative stress, which results in a higher production of antioxidant defense compounds (Pei et al., 2020), as observed here: reduced glutathione, phenols and DPPH (Table 3). Whan et al. (2016) found that F. oxysporum infection in Gossypium hirsutum increased the phenolic compounds concentration, as seen here. Regarding silicon, Hajiboland et al. (2017a) have also observed that when applied to Nicotiana rustica plants, the concentration of phenolic compounds increases, only when they are subjected to mechanical stress. In the plant, silicic acid binds with hemicelluloses, pectin and polyphenols forming cell walls, in addition to stimulating a higher concentration of these components and lignin (Babalar et al., 2016). This strengthens the plants against attack by pathogens.
Similarly, it has been suggested that Si role against the attack of F. oxysporum in banana crop was possibly due to the potentiation of the phenylpropanoid pathway (Fortunato et al., 2014), which is related to the production of phenolic metabolites such as flavonoids, lignin, etc. which are synthesized from L-phenylalanine that produces trans-cinnamic acid derived from PAL content (Hajiboland et al., 2017b; Hussain et al., 2021). This would explain the increase in flavonoid content in plants treated with ASi+Fol. Madany et al. (2020), for example, suggested that Si NP favored PAL activity, and thus to the increase in content of lignin. However, the results of this study do not show a difference with the application of ASi+Fol with respect to Fol in phenols and PAL (Table 3). On the other hand, treatment with Fol increased the PAL (Table 4) and also the content of total phenols respect T0 (Table 3).
It is known that F. oxysporum colonization can trigger defense mechanisms, for example, increased callose and lignin deposits in the cell structures or increased ROS and, consequently, greater antioxidant activity (Pei et al., 2020). Dorneles et al. (2017) got a higher CAT activity, as observed here (Table 4). However, CAT was not affected in the plants treated with ASi+Fol, possibly due to a low activity of this enzyme in H2O2 elimination (Dallagnol et al., 2015), as well as Habibi, (2015) also did not observe changes in CAT in canola under water stress. Which suggests less ROS concentration in this treatment, and therefore less stress (Hu et al., 2020), since in addition to low CAT activity, low concentrations of reduced glutathione and DPPH were also observed in ASi+Fol treatment.
As already mentioned, the infection of pathogens to plants induces an increase in oxidative stress, triggering antioxidant defense (Kang et al., 2014) producing antioxidant enzymes such as GPX and other antioxidant compounds. Silicon can also function as elicitor in plants and generates changes the defense-related enzymes activity and together with other mechanisms lead to induced resistance (Sakr, 2021; M. Wang et al., 2017). This explains the increase of GPX observed in this work in treatments with ASi or Fol (Table 4).
Likewise, SOD activity has a major importance in the defense against pathogens, since it regulates the concentration of ROS in cells (Hao et al., 2011; Sattar et al., 2020). It was found that increase SOD activity was caused by a higher generation of H2O2, conferred by Podospaera xanthii, in melon plants added with Si (Dallagnol et al., 2015), however, these results do not agree with those reported in this study (Table 4). In this regard, it has been reported that Si-treated plants exhibited reduction in SOD and CAT activities in Cercospora sojina infected soybean (Telles Nascimento et al., 2016). Which suggests a lower concentration of ROS in ASi+Fol treatment (Hu et al., 2020), in addition, other antioxidants (e. g. GPX) could also be involved in the elimination of ROS. In this case, the positive effect observed on the incidence and severity of Fol wilt may also be the result of the efficacy of other mechanisms, such as phytohormones synthesis and modification of gene expression.
Secondary metabolites
Since SA accumulation is key in the defense of tomato plants after the attack of Fol (Di et al., 2017) the observed increase is directly related to the defense system against Fol (Table 5). Salicylic acid exacerbated the systemic acquired resistance (SAR) conferred by Fol infection in Musa acuminata (Z. Wang et al., 2015). In a previous study, it has been observed that the application of Na2SiO3 showed lower concentrations of JA in Oryza sativa than mechanical stress treatment alone (Kim et al., 2014); similar findings to those obtained in this work (Table 5).
The results obtained here (Table 5) demonstrate that Fol infection triggers the defense mechanism of tomato plants, increasing the SA and JA generation (Jang et al., 2018; Vivancos et al., 2015). This can be caused by ROS accumulation such as H2O2 that induce the activity of the lipid hydroperoxidase and benzoic acid hydrolase enzymes, that stimulate the synthesis of jasmonic acid and that transform benzoic acid into salicylic acid, respectively (Dorneles et al., 2017; Fagerstedt et al., 2010). Silicic acid application can also induce the SA and JA biosynthesis (Jang et al., 2018), however, the intensity of the response is less than that caused by F. oxysporum, and it may take longer to appear as observed here (Table 5).
Gene expression
The PR1 and JA genes expression responses are derived from the increase in salicylic and jasmonic acids observed in treatments with Fol and ASi+Fol (Table 5). This is because SA and JA are hormones that regulate the expression of these defense responses related genes (Han & Kahmann, 2019). It highlights the production of PAL and various antioxidant compounds (Tables 3 and 4) involved in the ROS reduction.
The results show that Fol triggers the plant defense system, such as the expression of related genes. Although silicic acid application also induces SA and JA production (Table 5), these markers did not have a very strong impact on PR1 and JA genes expression (Figure 2), unlike other authors’ reports (Cai et al., 2009). Gulzar et al. (2021) cited that silicon amendment in Lycopersicon esculentum inoculated with Alternaria solani, had an up-regulation the PR3, LOXD and JERF3 (JA marker genes). Manivannan & Ahn, (2017) documented that Si treatment-induced resistance was manifested by activating the SAR, through the regulation of the jasmonic acid pathway and genes related in the hypersensitivity response. Therefore, the positive effect observed on the incidence and severity of Fol wilt may occur due to the regulation of other pathogenesis-related genes, or due to the increase in antioxidant compounds (Tables 2-4), or even structural modifications (Dann & Le, 2017; Vivancos et al., 2015).
The gene GAME1 is related to phytoalexins, which are mainly phenolic compounds that accumulate when infections by phytopathogenic fungi such as F. oxysporum appear, participating in the defense against them (Jeandet et al., 2002). However, the results showed an opposite effect with the inoculation of Fol, since this gene expression was repressed (Figure 3A). Opposite case, the clear effect on the expression of GAME1 gene by the silicic acid application is consistent with other authors’ reports, who found that production of flavonol phytoalexins in Cucumis sativus and momilactones in Oryza sativa, respectively, is stimulates by silicon (Fawe et al., 1998; Rodrigues et al., 2004). Therefore, the production of phytoalexins induced by the silicic acid application could have affected the progress of the incidence and severity of Fol wilt observed in tomato (Figure 1).
Overexpression of PAL5-3 is important to regulate Fusarium infection, since it leads to a greater accumulation of lignin and synthesis of salicylic acid to trigger SAR (Iqbal et al., 2005). Therefore, tolerance to this pathogen can be increased when these circumstances occur, as observed in this study (Figures 1 and 3B). Zvirin et al. (2010) demonstrated that PAL5-3 expression in Cucumis sativus increased as colonization of F. oxysporum progressed. In addition, Cruz et al. (2015) observed in wheat plants inoculated with Pyricularia oryzae an increase in PAL expression independently of the application of silicic acid, however the highest level of expression was in the silicic acid treatment. These results agree with the observed, since although all the treatments increased the expression of the PAL5-3 gene, the treatment of only silicic acid presented the highest overexpression (Figure 3B). Kuai et al. (2017) and Shetty et al. (2011) obtained higher transcripts of this gene when applying silicon; this led to an increase in lignin and phenolic compounds, reducing the severity of pathogens, similar to observed here (Table 3, Figure 1). These results indicate that indeed the application of silicon, in this case as silicic acid, increases the expression of the PAL5-3 gene. This translates into greater tolerance to pathogens such as Fol, through different mechanisms that can be biochemical (phenolic compounds) or mechanical as physical barriers (lignin).
These results seem to indicate that silicic acid application has a more pronounced effect on the expression of ET gene, in comparison with the expression induced by Fol (Figure 4A). Commonly the attack of pathogens, like Fol, in plants, triggers the production of antimicrobial compounds as well as stress hormones such as SA, JA and ethylene (Table 5, Figure 4A) (Islam et al., 2019).
However, silicon also confers the activation of genes involved with plant stress, specifically in the form of silicic acid, it induces defense reactions that improves the production of stress hormones (Fauteux et al., 2005). This was observed mainly with the ethylene related ET gene (Figure 4A). This finding agree with that of Van Bockhaven et al. (2015) who reported that silicon intervenes specifically in the ET signaling pathway. Similarly, Ghareeb et al. (2011) demonstrated that Si-induced resistance is mediated by the ET and JA signaling pathways in tomato plants infected with Ralstonia solanacearum. This suggests that in the case of tomato, the application of silicic acid increases tolerance to Fol (Figure 1) by regulating the genes related to phytoalexins (gene GAME1, Figure 3A) and the production of ethylene (Figure 4A).
In the case of ABA gene, apparently Fol induces a greater expression of this gene in comparison with the application of silicic acid (Figure 4B). Although the reports mention that the attack of pathogens in plants involves the production of ethylene (Cai et al., 2009; Fauteux et al., 2005), the results obtained in tomato indicated that Fol induces mainly the expression of the gene related to abscisic acid (Figure 4B). This can be derived from the antagonism between ABA and ethylene, in addition to the negative effect of ABA to pathogens tolerance (Mauch-Mani & Mauch, 2005). This is consistent with our results, since ABA gene expression increased with Fol inoculation in tomato especially at 73 dat, when the severity of pathogen is higher (more than 60%) (Figure 1B). While at 31 dat when no incidence of Fol wilt was found (Figure 1A), there were no significant effects in ABA gene expression (Figure 4B). Overall, it is clear that there is a complex interaction between PR1, JA, GAME1, PAL5-3, ET and ABA genes that result in different defense responses and tolerance to Fol in tomato (Anderson et al., 2004; de Lamo & Takken, 2020).
Conclusions
Stress by F. oxysporum in tomato plants increased antioxidant enzymatic activity and enzymatic activity antioxidant compounds in leaves, mainly vitamin C, total phenols, PAL, CAT and GPX. It was also observed that the inoculation of Fol in tomato increased the content of salicylic and jasmonic acid (247 and 81.3%, respectively). Additionally, Fol induces increased JA, GAME1, PAL5-3 and ABA genes expression, at 73 dat; however, it caused a lower concentration chlorophyll and flavonoids, and expression of PR1, JA, GAME1, PAL5-3, ET and ABA gene, at 31 dat.
Silicic acid application to tomato plants stressed by F. oxysporum (ASi+Fol), reduced the incidence and severity of Fol wilt (until 70 and 85 dat, respectively); since it increased the content of antioxidant compounds in leaves, mainly flavonoids (24.6%) and chlorophyll (248.6%). In addition to this, led an overexpression of genes associated with to salicylic acid, jasmonic acid, phytoalexins, phenylalanine ammonia lyase, ethylene and abscisic acid synthesis mainly (PR1, JA, GAME1, PAL5-3, ET and ABA), which, as a whole, increased the tolerance to Fol in tomato; this effect of silicic acid was observed in the first days of the transplant, since these genes were subsequently decreased, as well as reduced glutathione, DPPH, CAT and jasmonic acid was reduced.