Introduction
Soils are under threat worldwide (Drobnik, Greiner, Keller, & Grêt-Regamey, 2018). To minimize its degradation, it is important to measure their state of health. Soil science is considering a comprehensive assessment of soil quality for better decision making. The type of cropping system (monoculture, co-culture, or rotation) and the management regime (e.g., chemical vs. organic fertilization, irrigation, and tillage) can affect soil properties and microbial processes (Juhos, Czigány, Madarász, & Ladányi, 2019; Li et al., 2011; Nunes, van Es, Schindelbeck, Ristow, & Ryan, 2018; Olsson & Falkengren-Grerup, 2000). Differences in the distribution and quantity of litter inputs to the soil may affect the rate of organic matter (OM) degradation (Yavitt, Wright, & Wieder, 2004), soil enzyme activity (Baležentienė & Klimas, 2009; Yu, Ding, Luo, Donnison, & Zhang, 2012), and drive nutrient cycles, generally affecting soil fertility properties (Kaur, Sharma, & Puri, 2013; Peng et al., 2020). Some soil management factors such as organic or inorganic fertilization can induce changes in soil microbial biomass (Arrieche-Luna & Ruiz-Dager, 2010), soil CO2 emissions (Imer, Merbold, Eugster, & Buchmann, 2013), soil nitrification-rates (Alam et al., 2013) and soil organic carbon (Peng et al., 2020). All of these changes could modify the physical, chemical and biological soil properties (Udawatta, Kremer, Garrett, & Anderson, 2009), controlling plant productivity (Trasar-Cepeda, Leiros, & Gil-Sotres, 2008). Some changes in soil properties may be estimated or detected by bioindicators, which can reveal variations in soil-state faster than some chemical indicators (Gadzala-Kopciuch, Berecka, Bartoszewicz, & Buszewski, 2004). Bioindicators, such as PNRCM, can signify trends in soil and litter processes that affect crop productivity. The estimation of the heterotrophic respiration of soil is positively correlated with organic matter degradation (Yuste et al., 2007). The use of enzymes from bacteria, archaea and fungi has been widely proposed as bioindicators of soil biological functioning (García-Ruíz, Ochoa, Hinojosa & Carreira, 2008). Distinct differences in the metabolic functional capacity of microbial communities under different agricultural vegetative plant rhizospheres and soil depths were identified in a study measuring the activity of enzymes involved in nutrient cycling (C, N, P, and S; Gardner, Acosta-Martínez, Senwo, & Dowd, 2011). Additionally, changes in soil enzyme activities have been found to reflect temporal and spatial variations in microbial processes (Xu et al., 2020) and, consequently, in the soil mineralization processes (Akmal, Altaf, Hayat, Hassan, & Islam, 2012).
The use of extracellular enzymes produced by microorganisms as bioindicators has evolved as a strategy that aligns with nutrient and energy-supply demand (Burns et al. 2013). As a whole, enzymatic activities related to critical microbial processes of the soil, total acetyl esterases (FDA), phosphomonoesterase (pH, e.g., acid and alkaline phosphatases), phenoloxidases (POX, e.g., laccase) and peroxidases (PER, e.g. Mn peroxidase and Lignin peroxidase), cellulase (e.g. endoglucanase, β-glucosidase), urease and nitrogenase activities, are known to be related to the global biogeochemical carbon (C), nitrogen (N) and phosphorus (P) nutrient cycles (Sinsabaugh et al., 2008). FDA activity is the sum of several enzymes (i.e., lipase, protease, esterase), and has been extensively proposed as an indicator of overall microbial activity in soils (Green, Stott, & Diack, 2006; Schnürer et al., 1985). FDA activity in soils has been used to measure the effect of forest conversion into pastureland (Lopes, Salviano, Araujo, Nunes, & Oliveira, 2010), which showed higher sensitivity to changes in vegetation compared to measures of organic C. In addition, FDA has a strong positive correlation with biomass C, total organic C (Gajda, Przewloka, & Gawryjolek, 2013) and biomass N (Alvear, Urra, Huaiquilao, Astorga, & Reyes, 2007). Fungi and bacteria are the primary producers of cellulases and laccase enzymes, which are critical enzymes in lignocellulose decomposition. Cellulase activity controls cellulose hydrolysis, and it has been shown that the enzyme activity increased with organic matter concentration (Sinsabaugh et al., 2008; Wang et al., 2012). Laccase plays a principal role in the lignin-oxidation process and is also sensitive to soil phenolic-compounds (Diez et al., 2006). Phosphomonoesterases (acid and alkaline phosphatases), on the other hand, are responsible for organic phosphorous mineralization (Nannipieri, Giagnoni, Landi & Renella, 2011). Phosphorous, being the most limiting nutrient in soil ecosystems; makes phosphatases key enzymes for plant nutrition (Tarafdar & Jungk, 1987). Phosphatase activity was proposed to be highly related to soil fertility (Banerjee, Sanyal, & Sen, 2012); non-specific soil acid phosphatases become more abundant when enzyme cofactors (exchangeable calcium) are absent (Neal & Glendining, 2019). These enzymes are sensitive to different crops species (Nath & Samanta, 2012), phosphorous content, land use [higher activity in the forest than in the agricultural system], soil type [e.g., ultisol, oxisols (Acosta-Martínez, Cruz, Sotomayor-Ramírez, & Pérez-Alegría, 2007)] and organic matter concentration (Sinsabaugh et al., 2008).
Among the available information describing the bioindicators related to soil fertility, most of the studies have been carried out on monocotyledonous crops; because of their importance in human food and animal feed production (Avellaneda-Torres, Melgarejo, Narváez-Cuenca, & Sánchez, 2013; Gajda & Martyniuk, 2005). Despite the essential roles soil enzymes play in soil functioning and fertility, few studies have compared their use as bioindicators in tropical agroforestry.
Over the last 20 years, the tremendous economic value and exploitation of Cedrela odorata plantations have caused a decline in its natural populations. Conversely, commercial farms have expanded, bringing about much attention (Pennington Muellner, Wise, 2010) to sustainable management practices. However, little is known about the effects of different tropical agroforestry management regimens on soil biological properties and the use of soil enzymes as quantifiable bioindicators.
With this in mind, we evaluated the response of selected bioindicators (FDA, acid and alkaline phosphatase, laccase and PNRCM) associated to soil microbial activities occurring in two contrasting management regimes of Cedrela odorata: a monoculture (without irrigation nor chemical fertilization) and a co-culture of C. odorata combined with Citrus latifolia (Persian lime) (with irrigation and chemical fertilization). It should be noted that due to the different habitats of productive plant growth and similar adaptations to edaphic and climate conditions, (Aguilar-Luna Solorio-Sánchez, Hernández-Daumás, Huerta-Lwanga, Macario-Mendoza, 2011) proposed the use of C. odorata-Citrus latifolia combinations, citing this as a viable agroforestry system management practice.
We hypothesized that the bioindicators (FDA, acid phosphatase, alkaline phosphatase, and laccase activities, and microbial respiration, PNRCM) could help to discern the biological activities and quality of litter and soil under different management regimes of C. odorata plantations. It should be noted that the aim of this study is not to compare the biological activities between the plots, using them as treatments; the purpose is to relate the bioindicators values to the management regimes. The resulting information could lead to improved development of C. odorata trees. To have a holistic vision of the soil state, the organic matter (OM) composition, total N, available P and other soil micronutrients were also measured.
Objectives
The aim was to evaluate the use of soil bioindicators in the determination of the utility of two different Cedrela odorata forestry management systems. These soil bioindicators were associated to soil depth and C. odorata productivity attributes such as height and diameter.
Materials and methods
Study site location and agroforestry systems
The study site was in the municipality of Puente Nacional, in the center of Veracruz state, México, between 19° 20’ N and 96° 31’ O. Warm subhumid with rains in summer, medium humidity (61%), warm subhumid with summer rains, lower humidity (35%) and warm subhumid with rains in summer, with higher (4%) humidity (Instituto Nacional de Estadística, Geografía e Informática [Inegi], 2009). The site's climate is classified as oceanic -Cfb- (Köppen, 1936) with rainfall of approximately 900 mm to 1600 mm. The annual range of temperature is 20 °C to 26 °C. The study site has an altitude of 188 m a.s.l., and a Leptosol (55%), Vertisol (27%) y Phaeozem (17%) soil type (Inegi, 2009). The agroforestry systems studied were: 1) a monoculture of C. odorata with soil cover grass (neither irrigated nor fertilized), with an extension of ca. 5 ha and 2) a co-culture (irrigated and fertilized) of C. odorata combined with Citrus latifolia, with an extension of ca. 15 ha.
Sampling design and soil sampling
Each plantation system was divided into 10 plots of 50 m x 50 m. Three plots from each plantation were randomly selected to function as replicates. Soils under five randomly picked C. odorata trees were used for enzyme activity assessments, and two trees were randomly chosen to gauge carbon flux in each scenario. Composite samples of soil (1 kg each; 0-horizon) and litter (90 cm2; A-horizon) were taken at 1m away from the base of each tree and stored at 4 °C until analysis. Soil samples were taken from 0 cm - 5 cm and 5 cm - 10 cm depths, at the end of winter 2010.
Enzymes assays. Protein extraction
For enzyme activity quantification, total protein content was extracted according to Criquet, Tagger, Vogt, Iacazio, & Le Petit (1999) . 10 g of soil (or 5 g for litter) were weighed and ground, then, 90 mL of a CaCl2 solution of 0.2 molarity (M) was added, supplemented with 0.05% of Tween 80(polysorbate 80, polyoxyethylene sorbitan monooleate) as a non-ionic surfactant, and 6 g of polyvinylpyrrolidone (PVP). The mixture was stirred 2 h on an orbital shaker at 220 min-1, filtered with cotton gauze and centrifuged at 6000 min-1 for 20 min. Subsequently, the supernatant was passed through a 0.2 µm Whatman® filter supported by a vacuum pump. The filtrate was dialyzed for 24 h with a cellulose tubular membrane with polyethyleneglycol (PEG-8000 Ultrapure Usb, Cleveland, USA) as the dehydrating agent. The concentrated protein was recovered in 10 mL of 0.02 M, pH 5, Bis-Tris solution, and kept at -20 °C. The protein concentration was used to determine all the enzyme activities.
Acetylesterase activity (FDA) assay
The acetylesterase activity was quantified using the method proposed by Alarcón-Gutiérrez, Floch, Ruaudel, & Criquet, (2008) : 100 µL of the protein extract were taken and incubated in water bath at 37 °C for 1 h with 893 µL of phosphate buffer 0.2 M pH 7 and 7 µL of FDA as substrate (Sigma-Aldrich, USA). The reaction was terminated with 1 mL of water-acetone solution (1:1 v / v). The fluorescein released was quantified in a spectrophotometer (Genesis 10 UV, Thermo Spectronic, NY, USA) at 490 nm. Controls were made by adding the substrate at the end of the incubation (Schnürer & Rosswall, 1982) at denaturing conditions. All solutions were prepared with deionized and sterilized water. A fluorescein (Sigma Aldrich Chemical) standard curve (0 mg L-1 - 20 mg L-1) was made under the same conditions as the enzyme assays. Enzyme results were expressed in micromole of fluorescein released per minute (U) per gram of dry weight (U g-1) and units were converted to nano katals per gram (nkat g-1) using the following factor: 1 U g-1 = 16.6666667 nkat g-1.
Determination of phosphatase activity
For the determination of both alkaline and acidic phosphatase, the method of Tabatabai and Bremner (1972) was followed. For acid phosphatase, 100 µL of the extracted enzyme, 900 µL of 0.1mol sodium acetate buffer (pH 5) and 10 µL of p-nitrophenyl phosphate as substrate (Sigma-Aldrich, USA) were incubated at 37 °C for 5.5 h. For alkaline phosphatase, 900 µL of 0.1 mol pH 9 sodium hydroxide-glycine buffer was used. The reactions were stopped with 1 mL of 0.5 mol sodium hydroxide. The p-nitrophenol (p-NP) released was measured in a spectrophotometer at 412 nm (Thermo Spectronic Genesis 10 UV, NY, USA). A para-Nitrophenylphosphate (pNPP) calibration curve (0 mg L-1 - 20 mg L-1) was performed under the same conditions, and results were expressed in unit defined as micromole of pNP released per minute(U) per gram of dry weight (U g-1) and converted to nanokatals per gram (nkat g-1), using the following factor: 1 unit of enzymatic activity = 16.666 666 7 nkat.
Determination of laccase activity
Laccase activity in the extracts was measured using syringaldazine as substrate (Criquet et al., 1999). For kinetic measurements, 100 µL of the extracted enzyme, 990 µL of 0.1 mol phosphate buffer (pH 5.7) and 10 µL of a 5 mM syringaldazine (Sigma-Aldrich, USA) solution were mixed. The oxidation rates of syringaldazine to quinone were followed at 525 nm (with a molar attenuation coefficient ɛ = 65 000 mol cm-1) at 30 °C (Thermo Spectronic Genesis 10 UV, NY, USA). Initial velocities were determined from the linear parts of the curves. The results were expressed in units defined as micromoles of quinone formed from syringaldazine per minute (U) per gram of dry weight (U g-1) and converted to nanokatals per gram using the following factor: 1 unit of enzymatic activity = 16.666 666 7 nkat. Enzyme extracts from Pleurotus ostreatus were used as the positive control for laccase activities.
Soil and litter microbial respiration
Estimation of the potential net rate of carbon mineralization
Soil C potential net flux was determined through the PNCMR, according to Coleman et al., 1977 and Robertson, Coleman, Bledsoe, & Sollins, 1999). For this, 100 g of fresh soil were placed into tubes of polyvinyl chloride (PVC), the soil was wetted up to field water holding capacity by capillarity, and the tubes were introduced in a 1 L glass container, closed with an airtight lid, in which a vial with a CO2 trap was introduced (10 mL NaOH 0.5 nomal; Robertson et al., 1999). Soil samples were incubated at 25 °C for 30 days. During that time, measurements of CO2 captured in the traps were carried out every 48 h. For CO2 quantification, 5 mL of BaCl 1 normal were added to the traps, to precipitate the carbonates. Additionally, the residual NaOH was quantified by titration with HCl 0.5 normal. The final value was corrected for soil moisture, to obtain the soil dry weight values. Values were calculated as micrograms of C02 fixed each 24 h (µg day-1).
Size of trees
To associate the soil bioindicators with forest productivity, two variables were measured: 1) height of trees (in meters), measured with an altimeter (HAGA, Germany) and, 2) circumference of the tree at breast high (1.3 m), measured with a flexible tape measure (cm). Diameter at breast high (DBH) was calculated from the circumference, idealizing the transversal section of the tree as a perfect circle.
Soil physicochemical characterization
The following variables were measured: the amount of organic matter, determined by the method of Walkley-Black (Walkley, 1934) ; total nitrogen, by the Kjeldahl method; and available phosphorus, by the Brayʾs method (pH 7.2; Bray & Kurtz, 1945). The dry weight and moisture were determined by the gravimetric method, and pH was determined by the potentiometric method. Micronutrients, Ca, Mg, K, Na, Fe, Cu, Zn, and Mn were determined by atomic absorption spectrometry (Horwitz, 1997).
Statistical analysis
To determine the differences between the enzyme activity, microbial respiration, and size attributes of trees in culture systems, analyses of variance were performed with the management levels of the two plots (monoculture and co-culture), and three soil depths (surface litter, soil 0 cm - 5 cm and soil 5 cm - 10 cm) as main factors. Enzyme activity data was not normal (Kolmogorov-Smirnov P > 0.05) or homoscedastic (Levene, P > 0.05), so they were analyzed using the ANOVA rank transformation, as an analog of the nonparametric Mann-Whitney test (Conover & Iman, 1981). The response variables for each ANOVA model were the enzyme activities (FDA, APh and AlkPh; n = 18 and microbial respiration (n = 18). A t-test analyzed the attributes of tree size. This analysis was conducted using the statistical program R (R Development Core Team, 2010) with α = 0.05.
To identify the relationship between soil bioindicators and soil physicochemical properties in cedar plantations (monoculture and co-culture) at different soil and litter (0 cm - 5 cm and 5 cm - 10 cm), a Canonical Correspondence Analysis (CCA) with a permutation test (Pseudo F) of 500 iterations (α = 0.05) was conducted.
Results
Enzyme activities and PNRCM
Acetylesterase activity (FDA) was higher in litter than in the soil of both monoculture (litter; 0.38 nkat g-1 ± 0.3 nkat g-1 (; soil 0 cm - 5 cm depth = 0.05 nkat g-1 ± 0.03 nkat g-1) and co-culture (litter; 0.105 nkat g-1 ± 0.04 nkat g-1; soil 0 cm - 5 cm depth = 0.018 nkat g-1 ± 0.005 nkat g-1); and was higher in the litter of co-culture (1.05 nkat g-1 ± 0.17 nkat g-1) than in monoculture (0.67 nkat g-1 ± 0.01 nkatg-1; Fig. 1a; Table 1). In general, litter had more activities than soil; with more activities on co-culture (Fig. 1). However, no significant differences were found in the acetylesterase activity of soil between plots (P > 0.05; Fig. 1a).
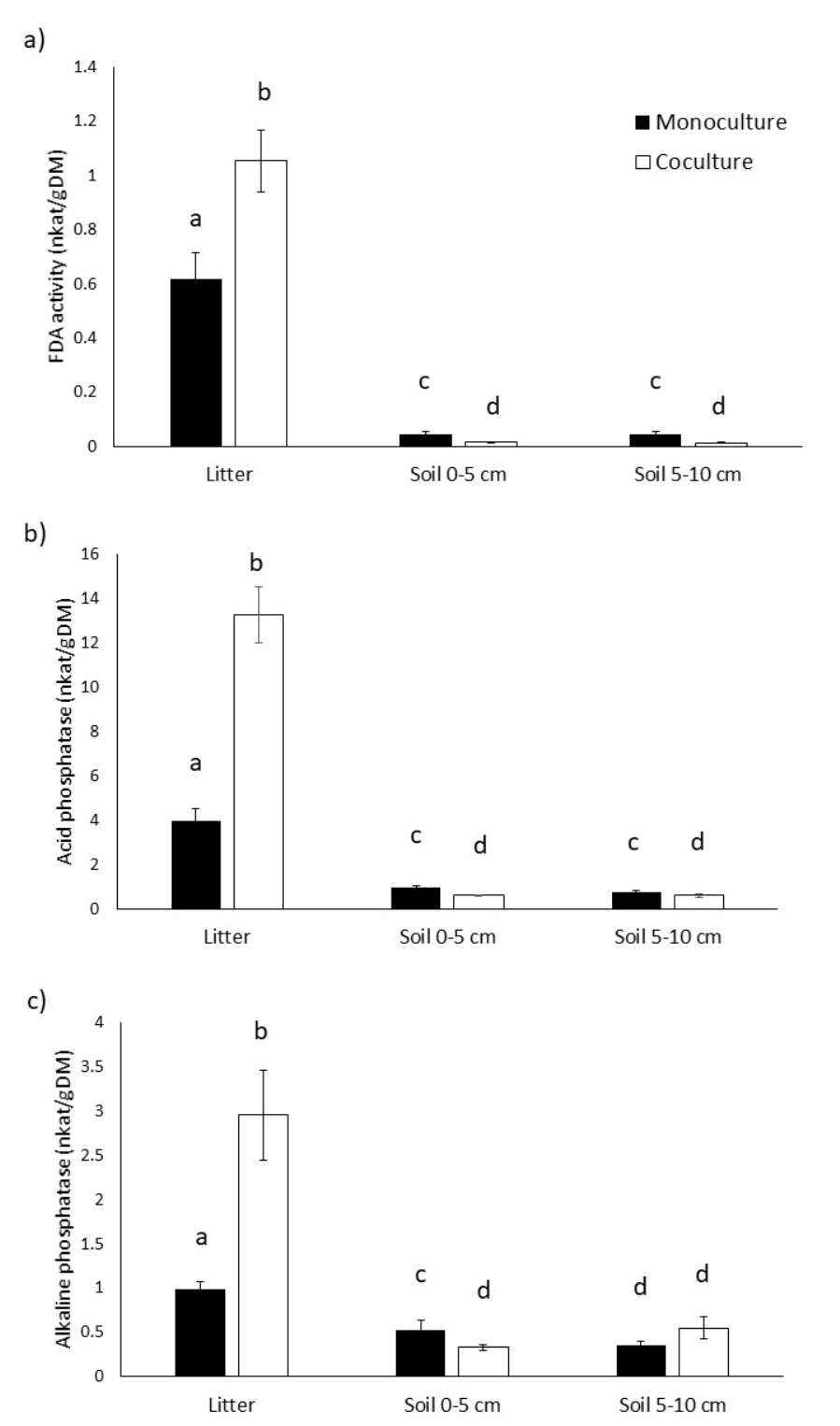
FIGURE 1 Values of enzyme activity registered in soil and litter of a monoculture and a co-culture of Cedrela odorata, a) FDA activity, b) Alkaline phosphatase activity, c) Acid phosphatase activity. Bars indicate the mean of data. Dispersion bars indicate standard error. Bars followed by different letters are statistically different P ≤ 0.05 (Tukey's test).
TABLE 1 ANOVA results of enzyme activities and the potential net rate of microbial respiration as a function of the culture system (Monoculture and co-culture) and depth.
Response variable | Source of variation | DF | SS | F | P |
Culture System (CS) | 1 | 1174 | 6.6 | 0.01 | |
FDA | Depth of soil (DS) | 2 | 32099 | 90.4 | < 0.001 |
CS*DS | 2 | 2250 | 6.3 | 0.003 | |
Error | 78 | 13854 | |||
Culture System (CS) | 1 | 629.8 | 2.6 | 0.1 | |
AlkPh | Depth of soil (DS) | 2 | 26475.8 | 54.1 | < 0.001 |
CS*DS | 2 | 3185.3 | 6.5 | 0.002 | |
Error | 78 | 19093.2 | |||
Culture System (CS) | 1 | 385.7 | 2.03 | 0.2 | |
AcPh | Depth of soil (DS) | 2 | 29527.4 | 77.7 | < 0.0001 |
CS*DS | 2 | 4642.4 | 12.2 | < 0.0001 | |
Error | 78 | 14828.6 | |||
Culture System (CS) | 1 | 0.67 | 0.04 | 0.8 | |
PNRCM | Depth of soil (DS) | 2 | 878.3 | 29.2 | < 0.0001 |
CS*DS | 2 | 0.58 | 0.02 | 0.9 | |
Error | 18 | 270.5 |
FDA = Acetylesterase activity; AlkPh = Alkaline phosphatase activity; AcPh = Acid phosphatase activity; PNRMR = Potential net rate of microbial respiration (net C mineralized). DF = degree of freedom, SS = the sum of squares, F = F-test to determine whether the variability between group means is larger than the variability of the observations within the groups, P = Probability value to determine the statistical significance (α = 0.05).
The second enzyme measured, Acid phosphatase (AcPh) activity, was higher in litter of co-culture (13.33 nkatg-1 ± 50 nkatg-1) than in monoculture (4 nkatg-1 ± 1.88 nkat g-1). In soil, differences between monoculture and co-culture were not significant (P > 0.05; Fig. 1b) for both depths (0 cm - 5 cm = 0.95 ± 0.1 nkat g-1 and 5 cm - 10 cm = 0.73 ± 0.07 nkat g-1 for monoculture; 0 cm - 5 cm = 0.66 nkat g-1 ± 0.05 nkat g-1 and 5 cm - 10 cm = 6 nkat g-1 ± 0.066 nkat g-1 for co-culture); however the AcPh was higher in the litter than in the soil of both managements .
A similar pattern was observed for Alkaline phosphatase (AlkPh). The activity was higher in litter than in the soil of both monoculture and co-culture; and was higher in the litter of co-culture (3.2 nkat g-1 ± 0.33 nkat g-1) than in monoculture (1.67 nkat g-1 ± 2.3 nkat g-1; Fig. 1c; Table 1). In general, as the soil depth increased the AlkPh activity decreased: monoculture [litter (1 nkat g-1 ± 0.08 nkat g-1 ); 0 cm - 5 cm (0.53 nkat g-1 ± 0.1 nkat g-1 ); 5 cm - 10 cm (0.35 nkat g-1 ± 0.05 nkat g-1)], co-culture [litter (3 nkat g-1 ± 0.5 nkat g-1 ); 0 cm - 5 cm (0.33 nkat g-1 ± 0.033 nkat g-1); and 5 cm - 10 cm (0.17 nkat g-1 ± 0.13 nkat g-1 )]. No significant differences (P > 0.05) were found in the AlkPh activity of soil between plots or between layers (Fig. 1c).
There was one enzyme, laccase, that neither monoculture nor co-culture registered activity in any sample analyzed. The method was validated using a positive control (enzyme extracts from Pleurotus ostreatus).
In the case of PNRCM, it does not differ as a function of the culture system (Table 1). However, the PNRCM had different intensities depending on the source: litter monoculture 546.6 µg day-1 ±119.8 µg day-1 was higher. For soil at 0 cm - 5 cm or 5 cm - 10 cm there was not statistically difference (P ≤ 0.05, Tukey's test;Fig. 2).
Attributes of cedar trees
Cedars from the co-culture system were larger in diameter and height (DBH= 49.01 cm ± 1.86 cm; height = 10.41 m ± 0.6 m) than trees of monoculture (DBH = 26.8 cm ± 1.4 cm; height = 6.05 m ± 0.4 m) showing significant differences in both parameters (F1,82 = 228.5, P < 0.0001 and F1,82 = 107.36, P < 0.0001, respectively, Fig. 3).
Soil physicochemical factors
The pH and soil moisture content did not vary between treatments/plots. The pH was slightly acidic for both (between 6.0 and 6.5; Table 2), and the moisture content was 21.78% ± 6.31% in the monoculture and 22.02% ± 4.88% in the co-culture plot. The organic matter content was higher in monoculture soil (3.88%, soil 0 cm - 5 cm) than in co-culture (2.53%, soil 0 cm - 5 cm). The amount of total nitrogen in monoculture was relatively high for the first soil depth (0.2%) and 50% lower for the second layer (0.1%) and did not change for the first and second depth from co-culture (0.144% and 0.154%, respectively). The available phosphorus in monoculture had low values in both depths (6.9 mg kg-1 and 4.5 mg kg-1, respectively), while higher values were found in co-culture for both depths (49.5 mg kg-1 and 40.4 mg kg-1, respectively: Table 2). The micronutrients Fe, Cu, Zn, Mn, and K were higher in soil from the co-culture than in soil from the monoculture, while B, and Ca were similar; Mg and Na were higher in monoculture soil (Table 2).
TABLE 2 Main macro and micronutrients of cedar soil plots in two different management systems.
Response variable | Monoculture (Soil depth) | Policulture (Soil depth) | ||
0 cm - 5 cm | 5 cm - 10 cm | 0 cm - 5 cm | 5 cm - 10 cm | |
pH | 6.4 | 6.3 | 6.45 | 6.46 |
Nt (%) | 0.209* | 0.145 | 0.144 | 0.154 |
OM(%) | 3.88* | 2.56 | 2.53 | 2.58 |
P Bray (mg kg-1) | 6.994 | 4.5 | 49.52* | 49.41 |
Fe (mg kg-1) | 19.2 | 19.22 | 20.82 | 23.91* |
Cu (mg kg-1) | 1.19 | 1.11 | 1.69 | 1.85* |
Zn (mg kg-1) | 0.41 | 0.1 | 0.81 | 0.95* |
Mn (mg kg-1) | 9.65 | 9.95 | 9.42 | 12.19* |
B (mg kg-1) | 0.28* | 0.18 | 0.27 | 0.22 |
Ca (g kg-1) | 5.96* | 5.88 | 5.74 | 5.32 |
Mg (mg kg-1) | 974.0* | 871.9 | 722.4 | 690.8 |
Na (mg kg-1) | 83.92 | 135.9* | 59.34 | 60.33 |
K (mg kg-1) | 325.9 | 228.7 | 748.6* | 485 |
The highest values are marked with an asterisk (*).
FDA = Acetylesterase activity; AlkPh = Alkaline phosphatase activity; AcPh = Acid phosphatase activity; PNRMR = Potential net rate of microbial respiration (net C mineralized). DF = degree of freedom, SS = the sum of squares, F = F-test to determine whether the variability between group means is larger than the variability of the observations within the groups, P = Probability value to determine the statistical significance (α = 0.05).
Canonical correspondence analysis (CCA)
The CCA summarized 75.11% of total inertia (variance) of the phenomenon in two new factors; F1 (66.92%) which is driven mainly by management systems variables (i.e., monoculture and polyculture) and F2 (8.19%), which is driven by soil depth (i.e. 0 cm - 5 cm and 5 cm - 10 cm; Table 3).
TABLE 3 Canonical correspondence analysis (CCA) results
F1 | F2 | |
Eigenvalue | 0.073 | 0.009 |
Restricted inertia (%) | 89.09 | 10.91 |
% aggregate | 89.09 | 100 |
Total Inertia (%) | 66.922 | 8.195 |
% aggregate | 66.922 | 75.117 |
The permutation test was significant (Pseudo F = 19.62; P < 0.0001), which indicates that response variables (bioindicators and soil physicochemical values) are linearly related with explicative variables (plot management system and soil depth). CCA plot (Fig. 4) shows enzymes activities of FDA, AcPh, and AlkPh occur mainly in the first soil layer, like PNRCM; and that these biological activities are closely related to percentage of organic matter (OM%). Soil enzyme activities are higher under monoculture compared to soils under polyculture. PNRCM is neutral for both management systems. Deeper soils (5 cm - 10 cm) were more abundant in mineral nutrients than the surface soils. Polyculture treatments contained higher concentrations of mineral nutrients compared to soils under monoculture.

FIGURE 4 Canonical correspondence analysis. F1 is given by management system variables, and F2 by soil depth variables; percentages of restrictive inertia explained are showed. Square markers represent the relative position of soil physicochemical properties (OM and Nt% and mineral nutrients) and soil bioindicators (PNRCM and enzymatic activities which are shown in bold) concerning the new principal components (F1 and F2).
Discussion
According to the results, the bioindicators measured, show that the employment of different management practices/regimes to produce C. odorata, differentially affects biological activities in the litter and soil, and therefore influences the soil physical and chemical parameters. The bioindicators used to evaluate changes in soil physical and chemical parameters, FDA and phosphatase activity (acid and alkaline) were sensitive to management system differences, mainly in the litter, while PNRCM values did not vary between management systems. AlkPh revealed differences in microbial activity depending on soil depth (i.e., litter, soil 0cm - 5cm, and 5cm - 10cm). Laccase activity was not identified in litter or soil, indicating low phenol-oxidase activity in this system, and indirectly, low fungal activity (Blackwood, Waldrop, Zak, & Sinsabaugh, 2007; Criquet et al., 1999; Zavarzina, Lisov, & Leontievsky, 2018). Although, laccase is a major enzyme used to assess lignocellulose decay, in this study the assay did not contribute useful information describing the biological state of the soil or litter, under the different C. odorata managements. Therefore, we do not recommend laccase enzyme as a general bioindicator of lignocellulose decay in cedar plantation soils. The use of laccase as bioindicator could, however, be useful, for more specific aims, like the assessment of phenolic degradation, as has been reported (Blackwood et al., 2007; Diez et al., 2006; Hernández et al., 2017).
FDA exhibited higher activity in litter compared to the soil in both management systems. This indicates higher microbial biomass activity in litter than in soil (Fig. 1a), mainly due to a more considerable amount of OM (Sánchez-Monedero et al., 2008; Schnürer, Clarholm, & Rosswall, 1985; Schnürer & Rosswall, 1982). This is indicative of the importance of litter as a reservoir of microbial activity, and that this is of increasing metabolic importance to cedar plantations, as has been reported for other ecosystems (Baldrian et al., 2012; García-Palacios, McKie, Handa, Frainer, & Hättenschwiler, 2016; Widmer, Shaffer, Porteous, & Seidler, 1999). FDA activity was higher in co-culture litter than in monoculture litter (Fig. 1a). According to this result, the co-culture, C. odorata with C. latifolia, management system, and irrigation/fertilization promotes more active microbiota (Caldwell, 2005; Green et al., 2006) than the C. odorata monoculture, which has different benefits for cedar development; for example, higher OM decomposition rate (Li et al., 2009), and the reestablishment of micronutrient banks (Nannipieri et al., 2003).
Soils collected under co-cultures at 0 cm - 5 cm depths had lower OM and Nt values, and higher concentrations of Fe, Cu, Zn, Mn and K than monoculture, at both depths (0 cm - 5 cm or 5 cm - 10 cm depths); however, monoculture showed higher levels of Na and Mg (Table 2). The higher concentrations of micronutrients found in co-culture soils is a consequence of the higher microbial activity in the litter, and the different biomass input (C. odorata + C. latifolia) added to the soil. Therefore, FDA is a suitable bioindicator of soil biological activity in the litter. FDA may also be used in the diagnosis developmental issues affecting tropical forestall plantations, which are sensitive to management changes, and thus may represent an estimation of the total microbial activity (Green et al., 2006; Schnürer & Rosswall, 1982).
PNRCM (heterotrophic respiration) was used as another bioindicator of general microbial activity. This bioindicator was sensitive to sample collected; litter or soil (Fig. 2) but showed similar values between management. PNRCM reached higher values in the litter, and decreased with soil, indicating that the decline of labile carbon sources between soil profiles is equal in both management systems. Moreover, soil OM (%) differed between cedar plots [as was previously mentioned (Table 2)], whereas PNRCM was not different. This indicates that the amount of soil OM in the two plots measured are different but have the same labile carbon mineralization rate (E.g. cellulose, hemicellulose).
However, monoculture could have a lower potential of recalcitrant carbon mineralization (e.g. lignin, humic acids), causing their accumulation. This may result in the soils from the monoculture to reach higher values of OM and Nt, but equal PNRCM values for the co-culture. This hypothesis suggests that soil from the co-culture has a different microbial community composition that promotes enhanced lignocellulose decay. This was also indicated in other studies that found higher vegetal biodiversity to be beneficial to soil microbial biodiversity (Guo-Chun et al., 2013; Navarrete et al., 2011; Paula et al., 2014). Additional studies are required to substantiate this reasoning. Another explanation could be that the estimation of heterotrophic respiration is a suitable method for the evaluation of short-term changes in soil carbon (Kutsch et al., 2010). In contrast, long-term changes in soil carbon chemical methods (repeated stocktaken) register all the “background” exacerbating the problem. Our results indicate that the use of PNRCM is suitable for the evaluation of heterotrophic CO2 respiration in tropical forestall plantations; and in combination with chemical data, could offer a significant holistic vision about the carbon sources, stocks, and consumption by microbial populations.
Similar to FDA findings, acid phosphatase showed higher activity in litter than soil in both management treatments and was the predominant form of phosphatase (4.04 more than alkaline phosphatase in co-culture´s litter, and 4.48 more in monoculture´s litter). The predominance of this enzyme form is likely a result of the soil pH (slightly acid; Table 2). Acid phosphatase and alkaline phosphatase showed higher values in litter from the co-culture compared to litter collected from the monoculture, which indicates higher phosphatase mineralization potential (Bini, Santos, Silva, Bonfim, & Cardoso, 2018; Krishnamoorthy, 1990; Nannipieri et al., 2011).
Phosphatase activity has been related to different factors (Della Mónica, Godoy, Godeas, & Scervino, 2018), such as chemical and environmental dynamics, for example, the presence of inorganic phosphorous in soil (Nannipieri et al., 2011), which inhibits the expression of PHO genes of phosphatase producers (Oshima, Ogawa, & Harashima, 1996). Although the soil co-culture had 7.13 times more P available (Bray) than the monoculture, there is no significant difference (P < 0.05) in the phosphatase activity that occurred in the mineral soil fraction of both systems. This could be a consequence of litter degradation due to the amount of available P in the soil resulting from the phosphatase activity in upper soil layers. Higher phosphatase activity in litter promotes higher available P in the soil, in addition to the P provided by inorganic fertilization. Our results indicate that the inhibition of phosphatase activity in soil by the amount of inorganic phosphorous infield is not proportional. However, a negative relationship between phosphatase activity and available P amount was observed in CCA (Fig. 3), similar to previous reports (Nannipieri et al., 2011; Bargaz et al., 2012). This study also shows that phosphatase mineralization is more active in litter than in soil (in cedar plantations) supporting the observation that phosphatase activity increases with organic matter concentration (Sinsabaugh et al., 2008; Wang et al., 2012).
Phosphatases are produced by diverse fungal (e.g. ecto, endo and arbuscular mycorrhizal fungi) and bacterial (Feng et al., 2002; Ho, 1989; Tarafdar & Chhonkar, 1979). Co-culture systems could promote better fungi and bacteria development, mainly because this system type offers better microclimate (protection of solar radiation; Krämer & Green, 2000) conditions for fungi and provides more diverse biomass inputs. All this contributes to OM decay, and P availability. We are in accordance with Banerjee et al. (2012) , who recommends the use of phosphatase activity in the assessment of soil fertility. In that study, phosphatase activities (acid and alkaline) were very sensitive to soil depth and plantation management, offering valuable information for soil diagnosis.
Regarding the vegetative development of the trees, cedars from co-culture were taller and grander in diameter than those of monoculture plot (Fig. 2). Possibly due to the interactions of the factors involved in the management regime, e.g., fertilization, irrigation and use of co-culture, those contribute to different carbon mineralization rate, microbial activity in the litter and a high enzymatic rate of OM decomposition. In turn, high enzyme activity would generate a more considerable number of available micronutrients to the trees. Our results are consistent with those reported by Aguilar-Luna et al., (2011) , who recommend the use of C. odorata-C. latifolia as a suitable production system for the tropics.
CCA revealed a positive relationship between soil biological activities and OM, and a negative association of phosphatase activities and available P amount. Micronutrients were not related to enzymatic activities or PNRCM in soil; also, co-culture soils reached higher values of micronutrients and lowered bioindicators values than soils under monoculture. Reflecting higher biological activity in the litter layer, as has been discussed above. This could be the cause of cedars trees had better dendrometric values (Fig. 3) on co-culture than in monoculture and better results on some soil nutrients (Table 2, Fig. 4). It is important to claim that both plantations are the same age, the same cultivation origin; sugar cane plantations and are under the same type of soil. CCA showed the importance of the first 5cm of soil depth (A horizon) for soil biological functions, because this layer has high activity for some bioindicators, like AlkPh and PNRCM, which contributes to micronutrient bank reestablishment at lower soil depths (e.g. 5 cm - 10 cm).
Conclusions
Sufficient sensitivity to soil spatial changes (i.e. litter to soil) and management systems (i.e. monoculture/co-culture) was achieved by FDA, phosphatase activities and PNRCM. The use of these bioindicators convey supplementary and valuable information to classic physicochemical indicators of fertility. We also conclude that, in this forest system; the biological activity in the litter is of critical importance for the release of micronutrients. Co-culture attained higher bioindicator values in the litter. Lower bioindicator values were detected in the monoculture soils, and higher micronutrients were found in the subsurface soil (5 cm - 10 cm) of co-culture treatments compared to co-noculture, which had lower micronutrient content and higher bioindicators values.
The Cedar-Persian lime system for forest plantations in tropic is recommended, due to better forest production and its promotion of higher biological activities in soil compared to cedar monocultures. However, these results are limited to plantations under the conditions here evaluated and must be taken with care; some results may be masked because the management type was not considered.