1. Introduction
Strontium ruthenate (Sr2RuO4) is a body-centered tetragonal crystal with a layered square structure for the ruthenium atoms [1]. Its normal state is described by a Fermi liquid, with three metallic conduction sheets in the Fermi surface (FS), namely the α, β, and γ sheets [2]. In addition, Sr2RuO4 is an unconventional superconductor with T c ≈ 1.5 K that strongly depends on non-magnetic disorder [3]. From the beginning, it was proposed that Sr2RuO4 is an unconventional superconductor with triplet pairing and some type of nodes in the order parameter for each sheet of the FS [4], where the symmetry of the superconducting gap is believed to break time reversal symmetry [5-7].
Although some authors consider that the γ sheet of the FS does not have nodes, several low temperature works have provided experimental agreement with nodes in the specific heat C(T), the electronic heat transport κ i (T) and directional ultrasound α j (T) measurements. These measurements resemble some kind of nodes on the FS including the γ sheet (see [8] and references therein for a review of the experiments with the first crystal samples of Sr2RuO4). Recently, novel experimental and theoretical advances continue to be carried out in the comprehension of the broken time-reversal symmetry superconducting state of Sr2RuO4. All recent and old studies continue to be crucial in order to explain the microscopic mechanism inherent to superconductivity in this compound ([9-12] and references there in).
In this work, we use a tight binding nearest neighbor expression for ξ
γ
(k
x
,k
y
) in order to model the γ sheet, which is centered at (0,0) in the first Brillouin zone, ξ
γ
(k
x
,k
y
) = −є + 2t[cos(k
x
a) + cos(k
y
a)] with hopping parameters (t,є) = (0.4,0.4) meV, and electron-hole symmetry. For the k dependence of the gap, we use the 2D tight binding expression corresponding to the Miyake-Narikiyo model [13] for a triplet state in the γ sheet, that is,
The nine points where the order parameter (OP)
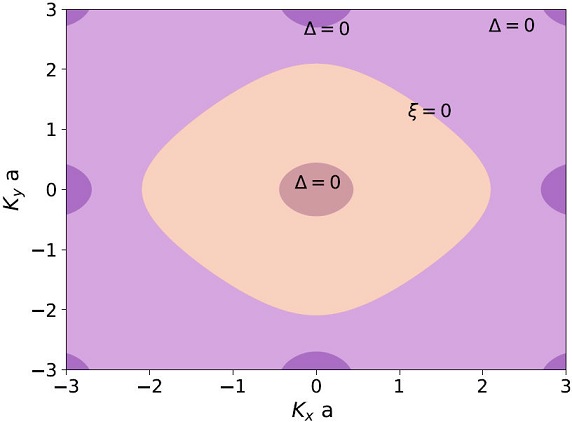
FIGURE 1 2D implicit plot of the tight binding anisotropic Fermi γ sheet ξ γ
(k
x
,k
y
) = 0 and the triplet superconducting gap with the localization of the nine points where
As noticed in Ref. [13], the gap on the γ sheet is very anisotropic and leaves a tiny gap around (0,±π) and (±π,0) points. According to group theory considerations, the imaginary OP has two components which belong to the irreducible representation E
2u
of the tetragonal point group D
4h
. It corresponds to a triplet odd paired state
In the following sections, we briefly report a visual numerical analysis of the MN model for the γ sheet on Sr2RuO4 at the phenomenological level (the microscopy mechanism is explained in their work [13]). We use a particular methodology [16]. First we vary the inverse strength parameter c from 0 to 1 and second, we vary the value of the parameter Γ+ from optimal to dilute doping in the function
2. From the unitary to the Born limit in triplet superconductors
We introduce the main equation for the elastic scattering involving the self-energy
Equation (1) describes the self-consistent renormalization in energy due to elastic scattering of superconducting pairs on non-magnetic atoms for the case of electron-hole symmetry in unconventional superconductors, i.e.
and the average over the γ sheet of the FS〈...〉 FS is performed with the tight binding expressions mentioned in the introduction, and according a numerical technique successfully used to fit experimental low temperatures ultrasound data with an accidental 3D point nodes model similar to the ZR model [20-22].
Finally, if electron-hole symmetry is not considered on the γ sheet, the other spin Pauli components
In the unitary limit, τ
−1(ω) has a resonance at zero frequency, that is,
In this section, we compute the solution of Eq. (1) by varying the parameter inverse to the strength c, and by fixing the value of disorder concentration Γ+ for two cases of physical interest. The first case is for a value of Γ+ = 0.3 meV, which resembles optimally doped values of impurities in experimental samples. The second case is for a dilute disorder concentration n imp , that is, Γ+ = 0.05 meV. In Sr2RuO4, Sr atoms in the lattice form an additional impurity level in the energy zone, thus, Sr atoms are part of the structure and also are the centers on which non-magnetic elastic scattering occurs.
Figure 2 shows the evolution

FIGURE 2 Evolution of the imaginary part of the scattering matrix from the unitary limit to intermediate regions for a value Γ+ = 0.30 meV.
In Fig. 2, non-magnetic disorder affects most strongly the low energy region up to 1.5 meV. We also see from Fig. 2 that in the normal state, for energies bigger that the gap value, the function
Figure 3 shows the evolution of
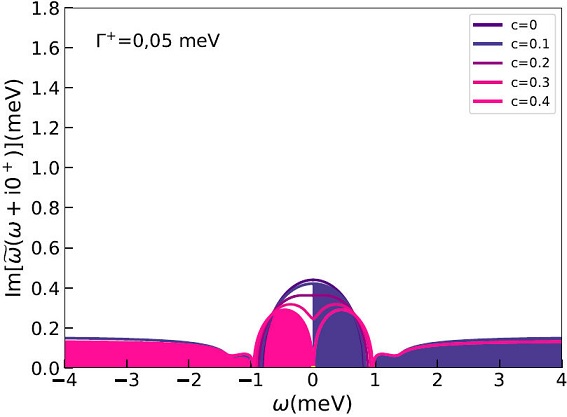
FIGURE 3 Evolution of the imaginary part of the scattering matrix from the unitary limit to intermediate regions for a value Γ+ = 0.05 meV.
Finally we observe in Fig. 3, an anomalous drop to zero¤ of the function
3. Inside the unitary limit, the visualization of the Miyake Narikiyo tinny gap in the γ sheet
In this section, we visualize the behavior of
The unitary regime is defined as the limit where the elastic scattering due to non-magnetic disorder is so strong that the mean-free path becomes comparable to the Fermi momentum k F , meanwhile in the Born metallic limit, the mean free path is much larger than the Fermi momentum k F . This means that normal state quasiparticles in the unitary region have an ill-defined momentum between elastic collisions. In this formalism, the signature of the unitary state is the resonance at zero frequency in the imaginary part of the scattering matrix.
Figure 4 shows the evolution of

FIGURE 4 Evolution of the imaginary part of the scattering matrix inside the unitary limit for nine values of the parameter Γ+ (meV).
In the left part of the Fig. 4, the MN tiny gap, that was numerically observed by using a density of states DOS analysis, is found in the
In order to compare the results, we performed an analysis using the same methodology for a lines nodes tight binding model for a High T c materials [16]. It allows us to state, that the case of the γ sheet tight binding analysis on Sr2RuO4, is much more sensitive to strong elastic scattering events and self consistency at low temperatures, since the unitary limit persists for most of the values used for the modeling parameters c and Γ+.
4. Conclusions
This communication was aimed at investigating numerically the behavior of the elastic scattering non-magnetic disordered averaged matrix
We found that the function
the behavior of the disordered matrix
The results were visualized in Fig. 4, the tiny MN gap was found for a Γ+ = 0:05 meV in disorder concentration. We end this short note pointing out that the MN model is very useful for setting up numerical studies in triplet superconductors such as strontium ruthenate, as we have demonstrated in this study.