1. Introduction
Different nuclear reactions such as elastic scattering, inelastic scattering, knock-out, pick-up, and stripping can occur when the nuclei interact with each other. Transfer reaction is one of them. Transfer reaction can be either a nucleon or a fewnucleon cluster form such as the α-particle. If it occurs in the form of α-particle transfer that can be assumed an elementary particle due to large binding energy [1], it is called the α-transfer reaction. The α-transfer reaction is generally observed in nuclei with α-cluster structure. It is evaluated in nuclear structure studies or α-clustering of nuclei. Also, it has an important place in the studies performed on astrophysical reactions such as the helium-burning and siliconburning [2,3].
The alpha transfer reaction 28Si(20Ne,16O)32S at 52.3 and 70 MeV was measured by using ICARE target facility of the Heavy Ion Laboratory of the University of Warsaw [4,5]. The experimental data were analyzed theoretically. However, the question of whether more compatible results with experimental data could be obtained leds us to do this study.
In the present work, we investigate the sensitivity to different density distributions of α-transfer reaction 28Si(20Ne,16O)32S. For this, we explore the entrance channel which is considered as a dominant cause of theoretical uncertainty in the analysis of the α-transfer reactions. In this context, we use ten various density distributions of 20Ne projectile. We compare the theoretical results and experimental data, and suggest the most suitable density distribution(s) for the α-transfer reaction 28Si(20Ne,16O)32S.
Section 2 shows the calculation procedure for the α-transfer reaction. Section 3 displays the density distributions of the 20Ne nucleus. Section 4 gives the results and discussion. Section 5 presents the summary and conclusions.
2. Calculation procedure
We consider the 28Si(20Ne,16O)32S reaction where the projectile 20Ne is treated as the composite system 20Ne = 16O + α. The α-particle is transferred to the target 28Si, and thus it leads to the formation of the composite target-like fragment in the exit channel¡ 32S = 28Si + α. The scheme of α-transfer reaction 28Si(20Ne,16O)32S is shown in Fig. 1.
For the theoretical analysis of 28Si(20Ne,16O)32S reaction, the various interactions should be considered; entrance channel¡ 20(Ne + 28Si), exit channel (32S + 16O), core-core (28Si + 16O), binding potentials¡ (16O + α and 28Si + α). The calculations related to 28Si(20Ne,16O)32S reaction require knowledge of interaction potentials for each partitions, which these potentials are described below. The cross-sections are obtained by using the code FRESCO [6].
2.1. Entrance Channel (20Ne + 28Si)
The potentials used for the entrance channel which is a dominant cause of theoretical uncertainty in the analysis of the α-transfer reactions play a significant role. With this goal, we examine the effect of the entrance channel on the cross section of the α-transfer reaction.
The nuclear potential of the entrance channel consists of the real and the imaginary potentials. To obtain the real potential, the double folding (DF) model is used via the DFPOT code [7] which is a very valid model in nuclear physics and many studies can be found in the literature [8-12]. The nuclear matter distributions of both projectile and target nuclei are very important in the DF calculations. Thus, the real part of nuclear potential is obtained by using ten different density distributions of the 20Ne nucleus assumed as a composite system, which these density distributions are explained in below. The imaginary part is thought as Woods-Saxon (WS) potential shown by
where W 0 is the depth, r w is the radius, and α w is diffuseness parameter. Thus, the nuclear potential can be written as
where NR parameter is the normalization factor which is used to increase the agreement between the data and the results.
The potential parameters used in the calculations with ten different density distributions are given in Table I.
TABLE I The potential parameters used in the calculations of the nuclear potential of the entrance channel.
Channel | Density | Energy (MeV) | NR | W0 (MeV) | rw (fm) | αw (fm) |
Ngo | 0.968 | 1.00 | 0.90 | 0.50 | ||
SP | 0.990 | 1.10 | 0.90 | 0.50 | ||
2pF | 0.955 | 3.10 | 0.90 | 0.50 | ||
G1 | 0.940 | 3.10 | 0.90 | 0.50 | ||
20Ne + 28Si | G2 | 52.3 | 0.930 | 3.10 | 0.90 | 0.50 |
J1 | 0.910 | 1.60 | 0.90 | 0.50 | ||
J2 | 0.970 | 1.00 | 0.90 | 0.50 | ||
M | 0.920 | 1.30 | 0.90 | 0.50 | ||
S | 0.970 | 1.10 | 0.90 | 0.50 | ||
HFB | 0.953 | 3.50 | 0.90 | 0.50 | ||
Ngo | 0.920 | 1.30 | 0.90 | 0.50 | ||
SP | 0.840 | 3.00 | 0.90 | 0.50 | ||
2pF | 0.680 | 3.00 | 0.90 | 0.50 | ||
G1 | 0.890 | 1.00 | 0.90 | 0.50 | ||
20Ne + 28Si | G2 | 70 | 0.850 | 2.00 | 0.90 | 0.50 |
J1 | 0.950 | 1.00 | 0.90 | 0.50 | ||
J2 | 0.863 | 1.00 | 0.90 | 0.50 | ||
M | 0.960 | 1.00 | 0.90 | 0.50 | ||
S | 0.923 | 1.00 | 0.90 | 0.50 | ||
HFB | 0.790 | 1.00 | 0.90 | 0.50 |
2.2. Exit Channel (32S + 16O)
The nuclear potential for the exit channel is assumed as the real and the imaginary potentials. In both cases the WS potential is used. Thus, the nuclear potential is formulated by
The parameters of the potentials used in the calculations are taken from Ref. [5], and are listed in Table II.
TABLE II The potential parameters used in the calculations of the exit channel, core-core and binding potentials.
Channel | V0 (MeV) | rv (fm) | αv (fm) | W0 (MeV) | rw (fm) | αw (fm) |
32S+16O | 100.0 | 1.22 | 0.50 | 30.0 | 1.25 | 0.40 |
16O+4He | 179.1 | 1.31 | 0.59 | 31.1 | 1.20 | 0.82 |
28Si+4He | 75.62 | 1.13 | 0.47 | 8.39 | 1.13 | 0.47 |
28Si+16O | 100.0 | 1.14 | 0.58 | 20.0 | 1.20 | 0.60 |
2.3. Core-Core (28Si + 16O)
The core-core potential describes the interaction between core and core nuclei. This potential is evaluated as the sum of the real and the imaginary potentials in the WS shape. As a result of this, the nuclear potential is the same with Eq. (3). The parameters of the potentials taken from Ref. [5] are given in Table II.
2.4. Binding potentials
The binding potentials for 28Si(20Ne,16O)32S reaction are considered as binding potential: entrance potential and binding potential: exit channel. The binding potential for the entrance potential is 16O + α, and the binding potential for exit channel is 28Si + α. The real and imaginary potentials for binding potentials are used in the WS form as similar to Eq. (3). In this context, the parameters used in the calculations are gotten from Ref. [5], and are shown in Table II.
3. Density distributions of 20Ne nucleus
3.2. São Paulo (SP), Two parameter Fermi (2pF), Jager 1 (J1), Jager 2 (J2), Moszkowski (M), Schechter (S) densities
The SP [15], 2pF [16], J1 [17], J2 [17], M [18], S [19] densities can be taken as the two parameter Fermi
where ρ 0i , R i and α i parameters are listed in Table III.
TABLE III ρ0i , Ri and αi values of SP, 2pF, J1, J2, M, S densities.
Density | ρ0 | R0 | α | Ref. |
SP | ρ0n=0.122086 | Rn=1.49N1/3-0.79 | αn=0.47+0.00046N | [15] |
ρ0p=0.0873779 | Rp=1.81Z1/3-1.12 | αp=0.47-0.00073Z | ||
2pF | ρ0n=0.0736291 | Rn=0.953N1/3+0.015Z+0.774 | αn=0.446+0.0072( |
[16] |
ρ0p=0.0759015 | Rp=1.322Z1/3+0.007N+0.022 | αp=0.449+0.0071( |
||
J1 | 0.153545 | 2.805 | 0.571 | [17] |
J2 | 0.162812 | 2.740 | 0.569 | [17] |
M | 0.16 | 1.15A1/3 | 0.50 | [18] |
S |
|
1.04A1/3 | 0.54 | [19] |
3.4. Gupta 2 (G2) density
The G2 density [22] is presented with different values of R 0i and α i given by
4. Results and discussion
The α-transfer reaction 28Si(20Ne,16O)32S at 52.3 and 70 MeV was examined for ten different density distributions of 20Ne projectile which consist of Ngo, SP, 2pF, G1, G2, J1, J2, M, S, and HFB densities. The changes with the distance (r) of the densities were presented in Fig. 2. The highest density in the center part was seen for the SP density while the lowest density was found for the HFB density. Moreover, the root mean square (rms) values of the density distributions were listed in Table IV.
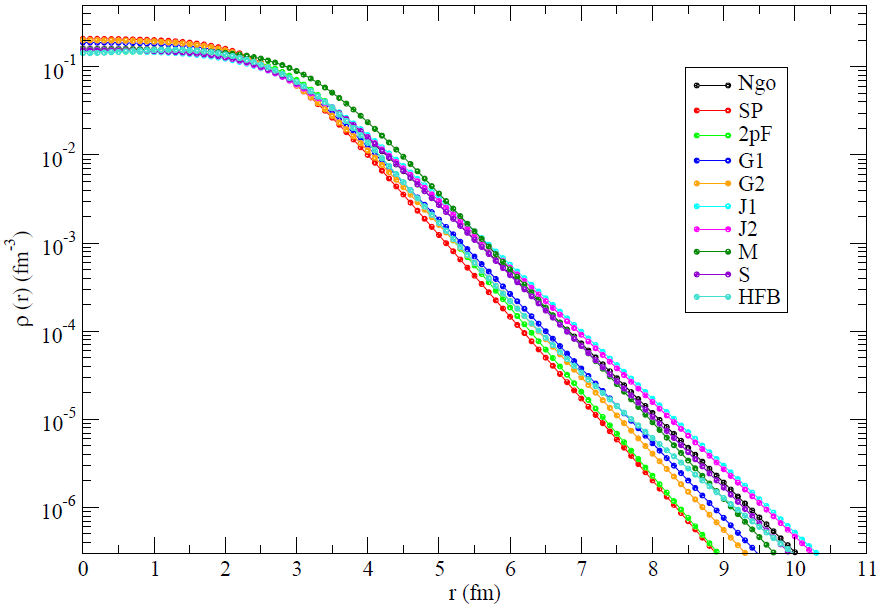
FIGURE 2 The changes with the distance (r) of Ngo, SP, 2pF, G1, G2, J1, J2, M, S, and HFB density distributions in logarithmic scale.
TABLE IV The rms radii for the Ngo, SP, 2pF, G1, G2, J1, J2, M, S and HFB density distributions.
Density distribution | rms radii (fm) |
Ngo | 2.955 |
SP | 2.665 |
2pF | 2.847 |
G1 | 2.789 |
G2 | 2.734 |
J1 | 3.037 |
J2 | 2.996 |
M | 3.049 |
S | 2.968 |
HFB | 2.842 |
We first calculated the cross-section of the alpha transfer¡ reaction 28Si(20Ne,16O)32 at 52.3 MeV by using ten different densities of 20Ne nucleus for the entrance channel. We compared the theoretical results with the experimental data in Fig. 3. We observed that the result of G1 density is slightly better than the result of 2pF density although the results of G1 and 2pF densities appear to behave close to each other. At the same time, the results of G1, G2 and HFB densities were found to be very close to each other up to 70 degrees, whereas it was seen that there was little difference at further angles. However, it can be said that very good results for G1, G2 and HFB density distributions are yielded although the results of G1 and G2 densities were slightly better than the HFB density.
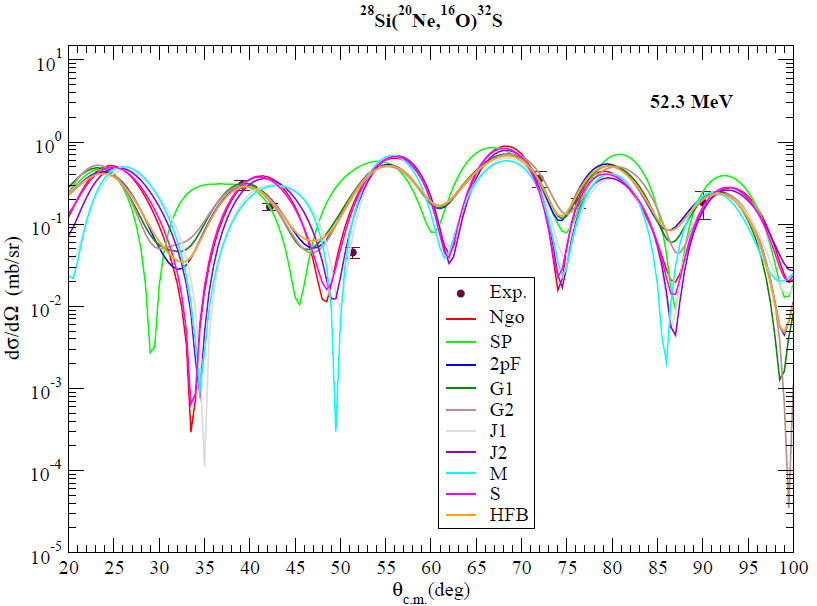
FIGURE 3 The cross-sections calculated for the α-transfer reaction 28Si(20Ne,16O)32 by using Ngo, SP, 2pF, G1, G2, J1, J2, M, S, and HFB densities in comparison with the experimental data at 52.3 MeV. The experimental data are taken from Ref. [5].
Then, we calculated the cross-section of the α-transfer reaction 28Si(20Ne,16O)32 at 70 MeV. We showed the results as compared with the experimental data in Fig. 4. We observed that the results of SP, G2, M and S density distributions are very good in defining the experimental data. As a result of this, we propose more than one alternative density distribution for the analysis of the alpha transfer reaction¡ 28Si(20Ne,16O)32.
In our study, we observed that cross-section results for all the density distributions are very sensitive to the N R constant used in the calculations of the DF model. When we examined the density distributions in this sense, we found the lowest value for J1 density and the highest value for SP density at 52.3 MeV, and the lowest value for 2pF density and the highest value for M density at 70 MeV. This shows that the real potential of the entrance channel is very effective in the analysis of the α-transfer reaction.
We also demonstrated the change with the distance (r) of the real parts of the nuclear potential for each density distribution in Fig. 5. In this context, the shallowest potential was obtained for the M density while the deepest potential was found for the SP density.
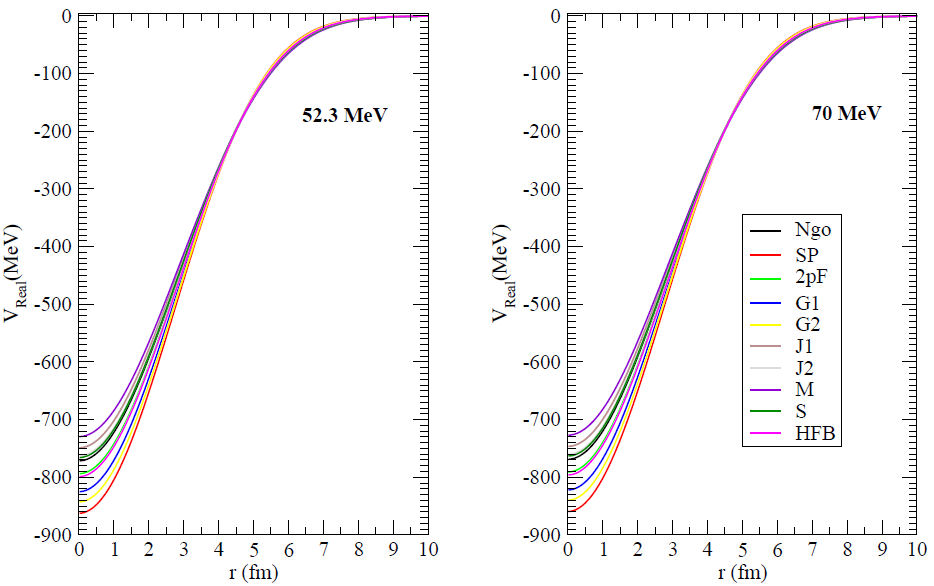
FIGURE 5 The changes with the distance (r) of the real potential depths calculated for Ngo, SP, 2pF, G1, G2, J1, J2, M, S, and HFB densities at 52.3 and 70 MeV.
Finally, we compared the literature results [5] with the best theoretical results for 52.3 and 70 MeV in Fig. 6. We observed that G1 and G2 results are better than the result of Ref. [5] at 52.3 MeV. Similarly, we noticed that the results of SP, G2, M and S densities are better than the result of Ref. [5] at 70 MeV. As a result of this, it can be said that the density distributions examined in this study have provided a significant correction on the cross-section of α-transfer reaction 28Si(20Ne,16O)32, and can be used as alternative density distributions in future studies related to α-transfer reactions.
5. Summary and conclusions
In this work, the α-transfer reaction 28Si(20Ne,16O)32 at 52.3 and 70 MeV was investigated for the entrance potential that is dominant in transfer reactions. The cross-sections were calculated for Ngo, SP, 2pF, G1, G2, J1, J2, M, S, and HFB density distributions of 20Ne projectile. It was observed that the G1 and G2 densities at 52.3 MeV, and the SP, G2, M and S densities at 70 MeV provided satisfactory results to the α-transfer experimental data. Also, it was observed they were better than both other density distributions and literature results. As a result of this, we can say that these densities will be alternative in the theoretical analysis of the α-transfer reactions. Additionally, we deduced that the entrance channel is very effective in the theoretical analysis of the¡ α-transfer reaction 28Si(20Ne,16O)32. We think it would be useful to apply this approach to other transfer reactions.