1. Introduction
In the past decades, there has been a significant interest in thermoelectric (TE) materials study. These materials are characterized by their capability to convert waste heat into electricity. Achieving a high-energy conversion efficiency in TE materials, however, has been the major obstacle for cost-effective applications [1-7]. The conversion efficiency of material for TE applications is characterized in terms of the dimensionless figure of merit ZT of the TE material, ZT = α2σT/κ, where α is the Seebeck coefficient, σ the electrical conductivity, T the operation temperature, and κ the total thermal conductivity (lattice and electronic contributions) [5,6]. Therefore, it becomes interesting to investigate the thermoelectric properties of compounds that already have low thermal conductivity.
One family of compounds that might satisfy such requirements is the rare-earth zirconates. With a chemical formula A2Zr2O7 (A = rare-earth element), these materials are ceramics that present either an ordered pyrochlore-type structure or a defective fluorite-type phase since the structure has a different formula than the fluorite structure (A,Zr)4O8 [7-9]. The rare-earth zirconates show interesting thermophysical properties. They present thermal and chemical stability, low thermal conductivity, and high thermal expansion, among other characteristics [10]. These promising attributes allow the rareearth zirconates to consider them excellent candidates for different industrial application, such as thermoelectric devices, thermal barrier coatings (TBCs), environment barrier coatings, etc. [11].
In order to consider rare-earth zirconates, or any other compound, as good prospects for thermoelectric applications, they must satisfy specific characteristics in their thermoelectric performance. The materials must have high electrical conductivity (from 0.01 to 0.6 S/cm), a high Seebeck coefficient (from 130 to 225 µV/K), and low thermal conductivity (from 0.5 to 0.75 W/mK) [12-15]. One of the important properties of the rare-earth zirconates that might offer a better figure of merit ZT is their low thermal conductivity. Thermal conductivities in these compounds have been reported to be considerably lower than the characteristic values obtained in yttria-stabilized zirconia [16-22], a compound commonly used in current TBC. Since the electrical and thermal conductivity values of the pyrochlorides, as well as the Seebeck coefficient, depend on the experimental conditions such as temperature and reaction time, as well as the concentration and type of the doped ion [14,23,24], it results interesting to explore further these compounds.
Pyrochlore oxides, in general, have been considered as promising candidates as solid-oxide fuel cell cathodes. The cationic disorder in these compounds favors the Frenkel defect formation responsible for the high-ionic conductivity and the oxide-ion diffusion in these materials [25,26]. In addition to the general properties of the Pyrochlore oxides and the particular characteristics of the rare-earth zirconates presented above to make such unexplored materials a promising alternative as possible cathodes in fuel cells.
In this paper, we investigate the thermoelectric properties of rare-earth zirconates. We are interested in obtaining polycrystalline A2Zr2O7 compounds with A = Pr, Nd, Sm, Gd, and Er via the solid-state reaction method. In particular, we study the heat-thermal conditions during sample preparation for which the compounds can be obtained. Electrical and thermal conductivities, as well as Seebeck coefficients, are determined as a function of temperature. We report the relationship between the structural and thermoelectric properties of the A2Zr2O7 compounds.
2. Materials and methods
2.1. Synthesis by solid-state reaction
Polycrystalline A2Zr2O7 samples (A = Pr, Nd, Sm, Gd, and Er) were synthesized by solid-state reaction. Before the reaction, the purity of the starting materials ZrO2 (Riedelde Haën, 99.9%), Pr2O3 (Aldrich, 99.9 %), Nd2O3 (Cerac, 99.9 %), Sm2O3 (Aldrich, 99.9%), Gd2O3 (Aldrich, 99.9%), and Er2O3 (Aldrich, 99.9%) was verified by X-ray powder diffraction (XRD). The stoichiometric amounts of reagents were mixed and ground in an agate mortar for 30 min in order to get a homogeneous powder [27]. The resultant A2Zr2O7 powders were compressed into pellets (13 mm diameter, 1.0−1.5±0.05 mm thickness) by applying a pressure of 3 tons/cm2 for 5 minutes under vacuum. After that, the pellets were heated in the air from room temperature up to 1400◦C at 10◦C/min, kept at 1400◦C for 72 h, and then cooled down to room temperature at 3◦C/min.
2.2. Characterization techniques
Thermogravimetric analysis (TGA) was performed in order to study the thermal behavior of each A2Zr2O7 compound. The analyses were carried out by using TA Instruments SDT Q600 equipment, from 25 to 1200◦C. XRD measurements were performed on an APD 2000 diffractometer with CuKα radiation (λ = 1.5406 Å) and a graphite monochromator. The XRD patterns were obtained at ambient temperature from 10◦ to 90◦ with a step size of 0.025◦ and a time per step of 15 sec. Rietveld refinement of the XRD patterns was performed by using the MAUD refinement software [28]. This program was developed by Wenk et al. [29] and Ferrari and Lutterotti [30] to analyze diffraction data in order to obtain the crystal structures of the samples.
Sample morphology and grain size were characterized by scanning electron microscopy (SEM) by using a Hitachi S3400N-II system equipped with an EDAX 9900 device that permits to determine the chemical composition of the samples by energy-dispersive X-ray spectrometry (EDS/EDX).
For the thermoelectric characterization, square-shaped compacts (10 × 10 × 0.5 mm) were prepared by using a 3-ton hydraulic press. Seebeck coefficient and electric conductivity were simultaneously measured in a high-precision SBA 458 Nemesis Netszch system. Measurements were performed from 300 to 900 K under a 10 sscm N2 flux and applying a current of 0.05 A. The heater voltage for Seebeck measurements was 1.0 V, whereas the temperature increment and the temperature difference threshold were 5 K/s and 15 K, respectively. Thermal conductivity was measured on a LFA 467 HyperFlash Netszch system equipped with a xenon flash lamp and an InSb detector. Experiments were also performed from 300 to 900 K, with a pulsed energy up to 10 J/pulse and a pulse width of 20−1200 µs.
3. Results and discussions
3.1. Thermal analysis
Previous to the synthesis process, the A2Zr2O7 compounds were examined by thermogravimetric analysis (TGA) to determine the temperatures at which the formation of subproducts takes place during the solid-state reaction process. The TGA curves of the samples are shown in Fig. 1. To simplify, the TGA thermograms are divided into three relevant regions according to the following reaction temperatures: 25−200◦C, 200−800◦C, and 800−1200◦C.
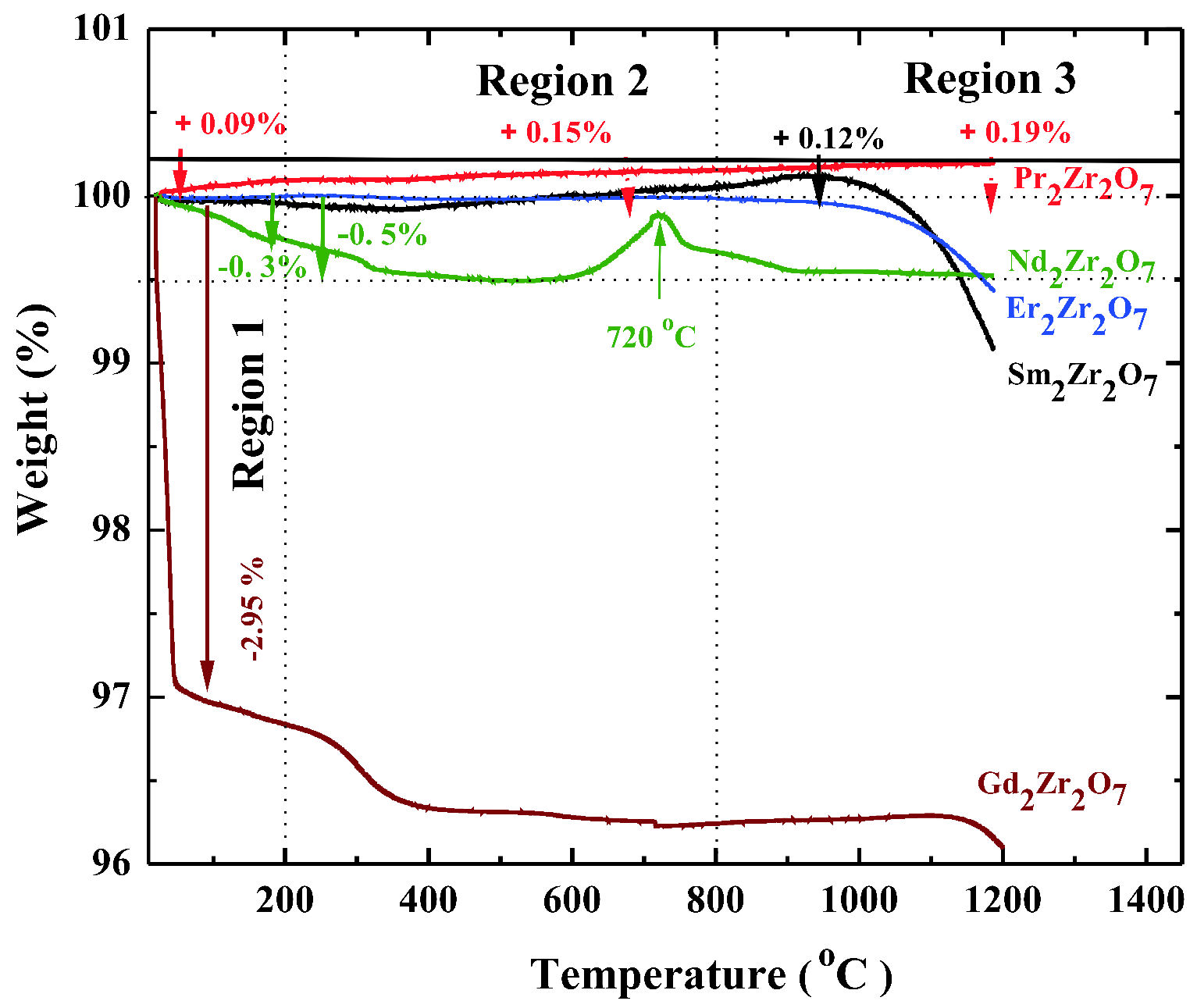
FIGURE 1 Thermogravimetric curves of the Pr2Zr2O7, Nd2Zr2O7, Er2Zr2O7, Sm2Zr2O7, and Gd2Zr2O7 samples.
In the first region, a weight loss of 0.05 - 2.95% was observed in the Sm2Zr2O7 and Er2Zr2O7, Nd2Zr2O7, and Gd2Zr2O7 samples, respectively. This result can be attributed to the humidity and possible water condensation on the samples’ surfaces. For the Pr2Zr2O7 sample, a gain of 0.09% was obtained, which can be explained by the possible reaction of the sample surface with the surrounding atmosphere.
In the second region, the Pr2Zr2O7 sample exhibits around 0.15% of mass gain, which can be attributed to a possible reaction with the surrounding atmosphere [31], whereas a negligible variation in weight was observed for the Pr2Zr2O7 and Sm2Zr2O7 samples. For the Nd2Zr2O7 and Gd2Zr2O7 samples, 0.5 and 3.5% of their weight loss, respectively, was detected between 200 and 400◦C. This can be related to possible oxidation caused by the decomposition at higher temperatures. Between 600 and 800◦C, a mass gain was obtained as a consequence of the reaction of the Nd2Zr2O7 sample with the atmosphere at 720◦C.
In the third stage, below 1000◦C, the TGA results show a mass gain of 0.12 and 0.19% for the Sm2Zr2O7 and Er2Zr2O7 samples, respectively. Above 1000◦C, no weight loss is presented in the Nd2Zr2O7 and Pr2Zr2O7 samples, which prove the elimination of all products before the formation of the desired compound.
3.2. Crystalline strucrure and phase composition
The XRD patterns of the A2Zr2O7 samples (A = Pr, Nd, Sm, Gd, and Er) are shown in Fig. 2. The results indicate the formation of Pyrochlore-type cubic phase with Fd3m space group (S.G. number 227). Main diffraction peaks, such as (222), (400), (331), (440), (662), (711), (800, and (662), and (840), are found in all samples. These peaks originate from the pyrochlorate structure [23-32,33]. Other minor peaks corresponding to raw materials were detected in some samples, such as ZrO2 (S.G. P21/a, no.14), Gd2O3 (S.G. I a3 , no.206), and Er2O3 (S.G. Fm-3m, no. 225) [34], indicating that minor quantity of reagents was not reacted.
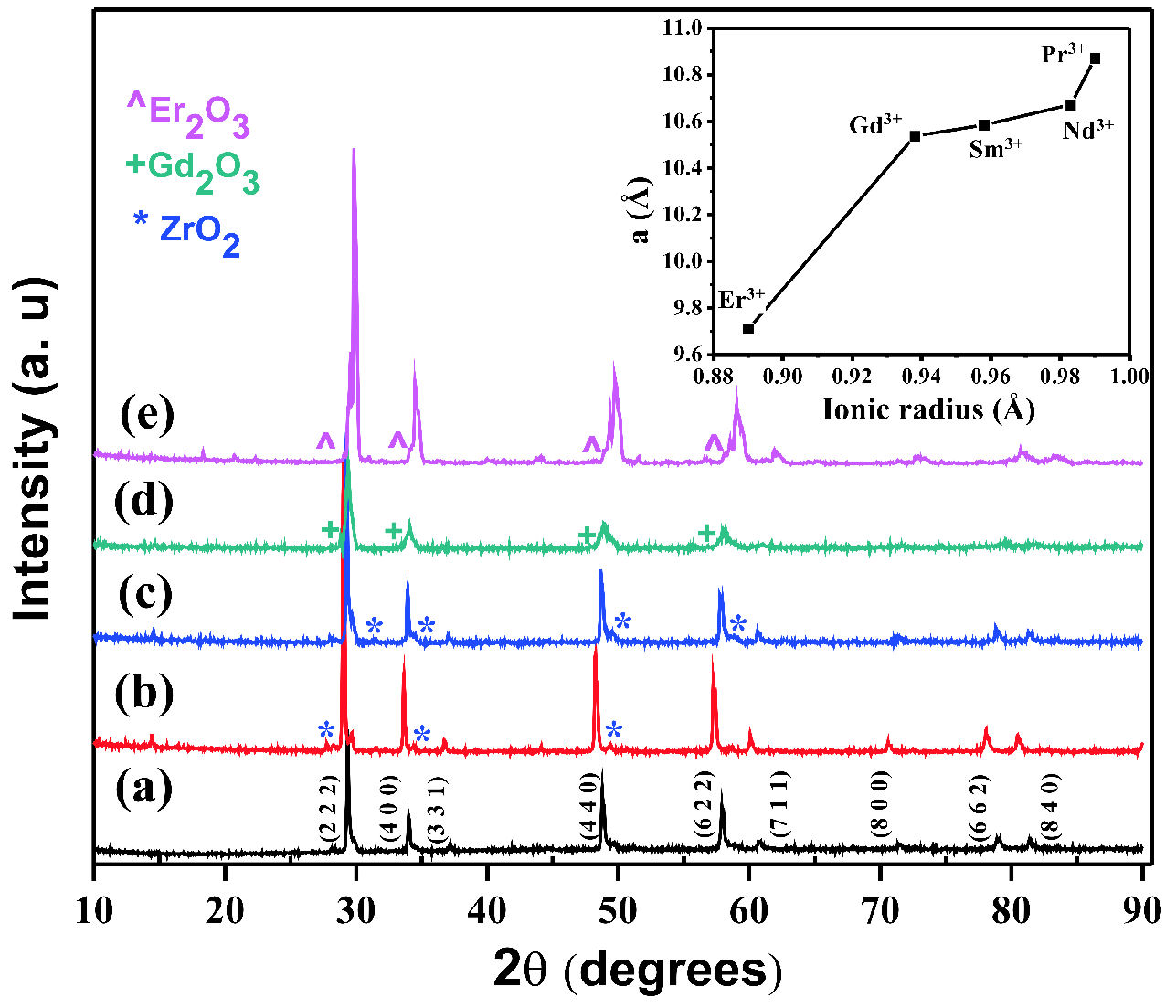
FIGURE 2 XDR patterns of the A2Zr2O7 samples sintered at 1400◦C. a) Pr2Zr2O7. b) Nd2Zr2O7. c) Sm2Zr2O7. d) Gd2Zr2O7. e) Er2Zr2O7. Inset: Cell parameter a as a function of the ionic radius.
The XRD patterns of the rare-earth samples were refined by using the MAUD software program [28]. The results showed a good agreement with the experimental pattern, confirming that the main phase presented in the samples is the cubic A2Zr2O7 phase. The values of Sig and Rwp after the refinement of the XRD patterns were within 1.35−3.23 and 6.184−23.868, respectively. Figure 3 shows the Maud refinement of the XRD data corresponding to the Gd2Zr2O7 sample.
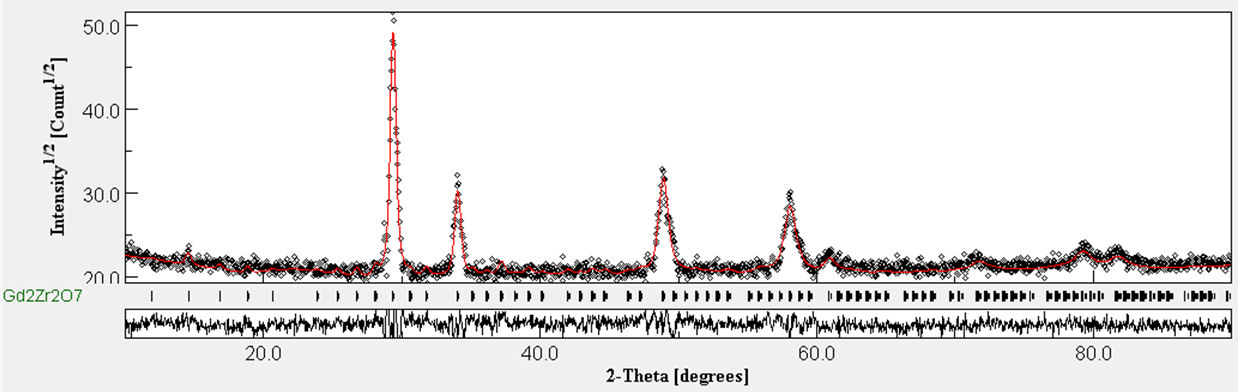
FIGURE 3 XDR patterns of the A2Zr2O7 samples sintered at 1400◦C. a) Pr2Zr2O7. b) Nd2Zr2O7. c) Sm2Zr2O7. d) Gd2Zr2O7. e) Er2Zr2O7. Inset: Cell parameter A as a function of the ionic radius.
The unit cell parameter A of the samples was calculated through structure Rietveld refinement. The inset of Fig. 2 shows the variation of the cubic cell parameter A as a function of the ionic radius (IR) of the rare-earth elements. An increase in the unit cell with an increase in IR of the rare-earth elements (IR(Er3+) = 0.890 Å < IR(Gd3+) = 0.938 Å < IR(Sm3+) = 0.958 Å < IR(Nd3+)= 0.983 Å < IR(Pr3+) = 0.99 A [35]) indicates the successful incorporation of the˚ rare-earth cations into the cubic structure durin the solid-state reaction.
3.3. Morphology and chemical composition
SEM micrographs of the samples heated at 1400◦C are shown in Fig. 4. The Pr2Zr2O7, Nd2Zr2O7, and Sm2Zr2O7 compounds show the formation of microcrystals with a size that varies between 0.7 and 2.4 µm. Moreover, it can be observed that the form of some grains are well defined, while all others form agglomerations (Figs. 4a), 4b), and 4c)).
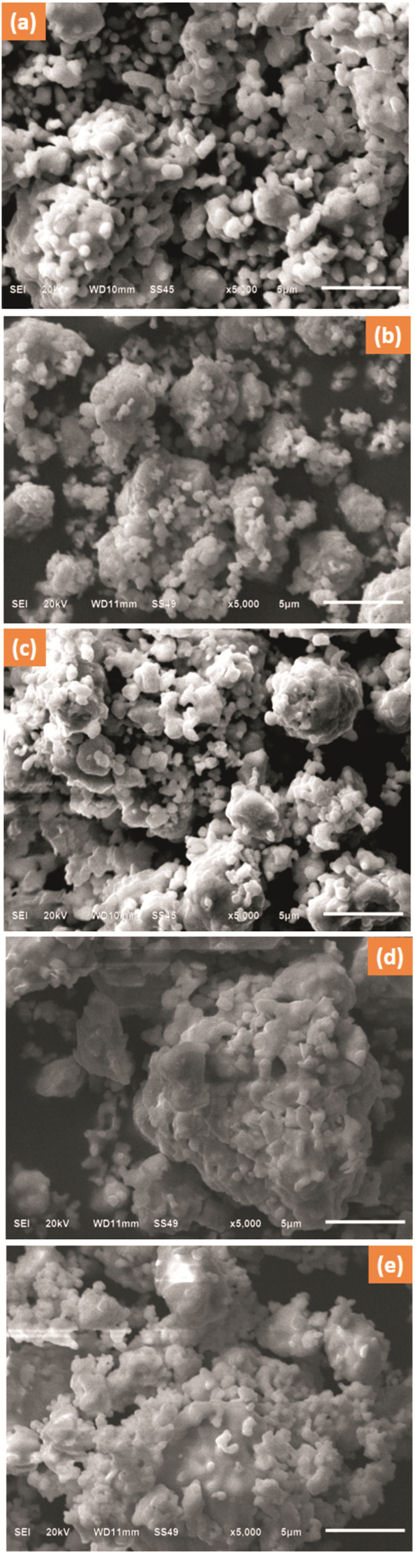
FIGURE 4 Micrographs of the a) Pr2Zr2O7. b) Nd2Zr2O7. c) Sm2Zr2O7. d) Gd2Zr2O7, and e) Er2Zr2O7 compounds prepared at 1400 ºC.
In the Gd2Zr2O7 and Er2Zr2O7 samples, the grains have sizes between 0.7 and 4.7 µm [Figs. 4d), and 4e), respectively]. Our results are consistent with the work given by Lucuta et al. [37], where they attribute these values to the high temperature used during the synthesis. Also, it can be observed in the figures that all samples are porous. Furthermore, the pores seem to accumulate at the grain boundaries. As indicated in other works, both porosity and grain size are considered important parameters that have a significant effect on the ferroelectric properties of the ceramics samples [14-16,36,37].
3.4. Thermolectric properties
The temperature dependence of the Seebeck coefficient S of each A2Zr2O7 compound (A = Er, Gd, Sm, Nd, and Pr) is presented in Fig. 5a). Positive and negative values of S are observed in the measured temperature interval, indicating that both conduction carriers, holes and electrons, are involved in the transport processes. Between 372 and 673 K, the absolute values of S are quite low in all samples. At temperatures higher than 673 K, the Seebeck coefficients of the Nd2Zr2O7, Sm2Zr2O7, and Pr2Zr2O7 samples slightly increase as temperature increases, indicating the semiconducting nature of these rare-earth zirconates. In the Nd2Zr2O7 sample, however, a maximum is observed at 773 K, followed by a drop. In contrast, S is kept almost constant for the Er2Zr2O7 sample. Figure 5b) shows the values of S extracted from Fig. 5a) at the fixed temperature of 773 K. This graph permits us to appreciate better that there exists a dependence of S on the ionic radius of the rare-earth elements A. This result is consistent with the work given by Moon et al. [16], where it was reported that S not only depends on A but also on the degree of structural distortion that determines the electronic localization-delocalization transition.
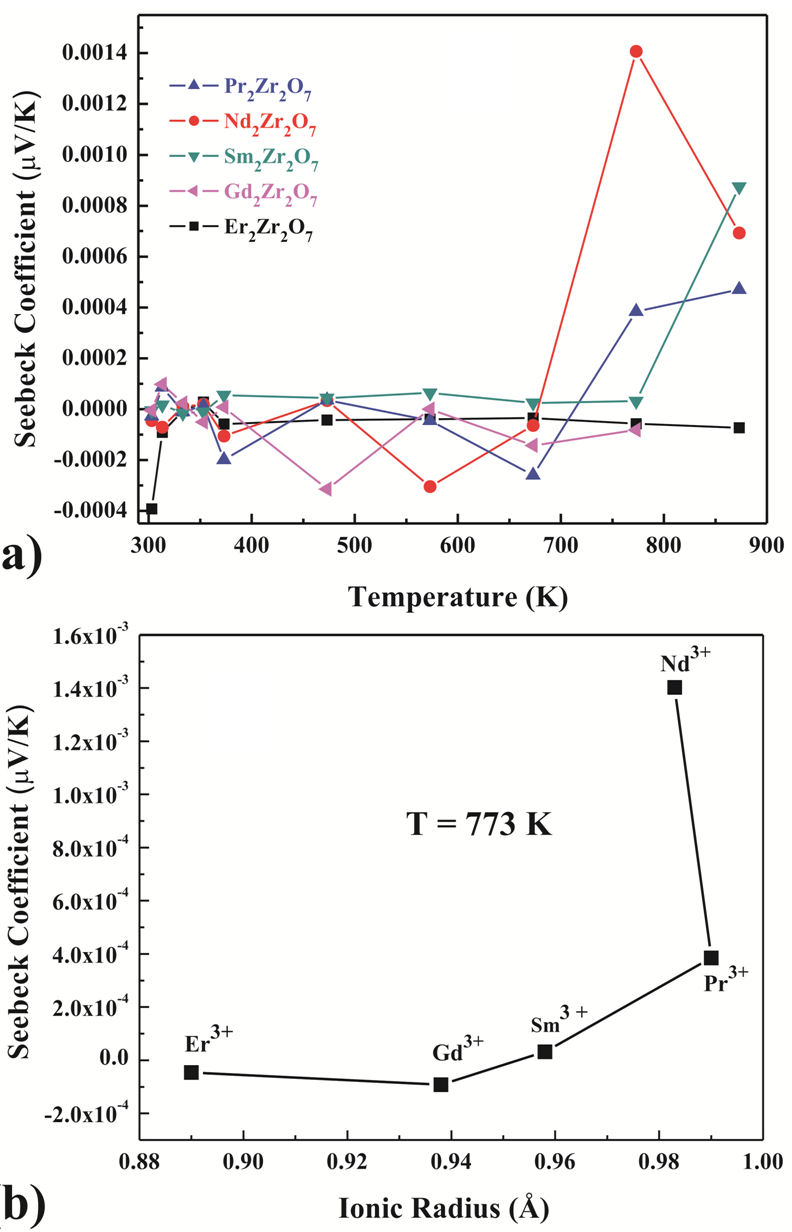
FIGURE 5 a) Seebeck coefficient of the A2Zr2O7 compounds (A = Er, Gd, Sm, Nd, Pr) vs. temperature. b) Dependence of the Seebeck coefficient on the ionic radius of the rare-earth elements at 773 K.
Figure 6 shows the electrical conductivity as a function of temperature. Low values of electrical conductivity are observed in all samples at temperatures larger than 400 K. This result can be attributed to the imperfections given by the high porosity observed in the SEM images (see Fig. 4) and to the structural disorder commonly presented in these materials [15]. In addition, it is well known that the electrical conductivity decreases with the number of charge carriers due to the filling of the valence band as a consequence of the doping. Besides, the additional electrons that are provided by the substitutional rare-earth solute cations (Pr3+, Nd3+, Sm3+, Gd3+, and Er3+) and replace the zirconium (Zr4+) sites are expected to shift the Fermi level from the valence band toward the middle of the bandgap [38]. It is also expected that the larger radius ratio (Pr > Nd > Sm > Gd > Er) produces higher defect-formation energy [39-41] that resist the structural transformations in the different compounds, giving rise to a low electrical conductivity.

FIGURE 6 Electrical conductivity as a function of temperature for the A2Zr2O7 compounds (A = Pr, Nd, Sm, Gd, Er).
Figure 7a) shows the temperature dependence of the thermal conductivity. Besides the Gd2Zr2O7 sample, the thermal conductivity of all samples monotonically decreases with temperature. For the Er2Zr2O7 sample, the thermal conductivity goes from nearly 1.2 W/mK at 300 K to 0.8 W/mK at 773 K. For the remaining samples, lower values of the thermal conductivity are obtained. Similar results were reported by Jie and collaborators [23], where the decrease in temperature of the thermal conductivity could be associated with the improvement in the efficiency of the thermal barrier coating compounds [23,24-42]. Figure 7b) shows the values of the thermal conductivity extracted from Fig. 7a) at the fixed temperature of 473 K. A dependence of the thermal conductivity on the ionic radius of the rare-earth elements A in A2Zr2O7 is clearly observed in the graph. A nearly monotonical behavior is obtained. The largest value of the thermal conductivity is obtained in the Er2Zr2O7 sample, the rare-earth zirconate with the total substitutional solute cation that has the lowest ionic radius, Er3+, whereas the lowest value is obtained in the sample with the largest ionic radius, Pr3+.
4. Conclusions
In summary, the thermoelectric properties of rare-earth zirconates A2Zr2O7, prepared by solid-state reaction with A = Pr, Nd, Sm, Gd, and Er, were studied. Once the samples were sintered at 1400◦Cduring 72 h, the XRD analyses showed the formation of polycrystalline Pr2Zr2O7, Nd2Zr2O7, Sm2Zr2O7, Gd2Zr2O7, and Er2Zr2O7 phases, respectively, with a cubic cell (space group Fm3m) and traces of raw materials. The chemical composition of each sintered sample, obtained by energy dispersive X-ray spectrometry (EDS/EDX) analysis, confirmed the formation of the respective desired stoichiometry with a small remaining quantity of the starting materials. Scanning electron microscopy (SEM) showed that all A2Zr2O7 samples present porous surfaces.
The Seebeck coefficient S measured at different temperatures in all A2Zr2O7 samples showed positive and negative values, indicating that both conduction carriers, holes and electrons, are involved in the transport processes in these rare-earth zirconates. At fixed temperatures, it was found that S increases with increasing the radius of the rare-earth ions A in the A2Zr2O7 compounds. The electrical conductivity, measured in the same temperature interval, showed low values in all A2Zr2O7 samples and was attributed to the imperfections given by the high porosity observed in the SEM images. Most interesting resulted from the thermal conductivity measurements. First, in most of the samples, the thermal conductivity monotonically decreased with temperature, where the Er2Zr2O7 sample showed the largest values and the Pr2Zr2O7 sample showed the lowest value. Second, it was found that the thermal conductivity decreases almost monotonically with the ionic radius of the rare-earth elements A in the A2Zr2O7 samples.
All these results indicate that rare-earth zirconates could be good candidates for thermoelectric applications. The substitutional rare-earth solute cations A in A2Zr2O7 could be used as a tuning parameter. More studies in these compounds, however, must be performed, particularly those on conversion efficiency. Besides the results suggest that rare earth zirconates could be used as a promising alternative for cathodes in solid-oxide fuel cells.
Author Contributions
The authors contributed to the synthesis and the characterization of the materials, like the data analysis. All authors discussed the results and contributed on the final manuscript.