1.Introduction
Currently, nanoparticles have various applications, including bringing drugs to specific targets (e.g. cancerous tissues 1). It is called a composite system, which is made up of cisplatin 2 and the silica nanoparticle (SiO2) 3, It is important to mention the interaction between the surface of the nanoparticle and the drug since the latter will synergize and effect the apoptosis process efficiently when it is internalized in the cancer cell 1,4. The composite system, when deposited in the cancerous tissue, is irradiated with electrons whose energy is 5 keV and knowing that the platinum atom has a great value for the photoabsorption cross-section and to a lesser degree for the oxygen atom. Giving thus, different types of interactions and emission of various responses that are generated in the platinum atom, such as characteristic X-ray emission, braking X-ray, photoelectron, Compton electron, and Auger electron. The Auger electrons are produced from a relaxation effect of excess energy in the atom after an electron from the innermost layers (K, L, M, N) of the atom platinum 5 has been ejected; these very low-energy, short-range electrons, less than 200 nm in human tissue 6, penetrate and interact in intracellular water.
Thus, the deposition of the energy of ionizing radiation (Auger electrons) in intracellular water establishes the formation of reactive oxygen species (ROS). The reactive oxygen species are broadly defined as oxygen-containing, reactive chemical species. There are two types of ROS: free radicals, which contain one or more unpaired electron(s) in their outer molecular orbitals, and non-radical ROS, which do not have unpaired electron(s) but are chemically reactive and can be converted to radical ROS. A free radical can be defined as an atom o molecule containing one or more unpaired electrons in valency shell or outer orbital and is capable of independent existence. The odd number of the electron(s) of a free radical makes it unstable, short-lived, and highly reactive. Because of their high reactivity, they can pull out electrons from other compounds to attain stability. Thus, the attacked molecule loses its electron and becomes a free radical itself, beginning a chain reaction cascade which finally damages the living cell 7.
This work simulates the production of reactive oxygen species generated when Auger
electrons react with intracellular water. To do this, the TOPAS-nBio 8-10 code was used, which was efficient in quantifying
the amount of ROS as a function of time and the energy spectrum of the Auger
electrons. The reactive species
Intracellular water was modeled as a very large spherical volume of water compared to the composite system (SiO2 nanoparticle + cisplatin); the cisplatin-surface interaction of the nanoparticle was not considered, nor was the radiation generated from the oxygen atoms taken into account (from nanoparticle and cisplatin). It should be mentioned that the simulation with the TOPAS-nBio code can be carried out with various sources of ionizing radiation, for different geometries and materials, thus showing its versatility and effectiveness, see Appendix A. This work is divided as follows: in Sec. 2, the formation of reactive oxygen species (ROS); in Sec. 3, geometric design of the physical system and simulation (TOPAS-nBio); in Sec. 4, the results and their discussion are presented; Section 5 presents the conclusions. In Appendix A, the Monte Carlo computational simulation (TOPAS-nBio code) is presented.
2.Formation of reactive oxygen species
The deposition of the energy of ionizing radiation (Auger electrons) in intracellular water, leads to the formation of reactive oxygen species (ROS). As a result of the radiolysis of water, the biological effects are derived, to a large extent, from the action of ionizing radiation on water molecules due, on the one hand, to its high presence in living beings, and on the other, to the fact of exercising its function as a solvent for other molecules or macromolecules in the biological system where important chemical reactions take place.
The action of radiation on water (radiolysis of water, a process that occurs between 10-12 and 10-6 seconds) is a sum of complex processes that can be simplified in two stages: the molecular decomposition of water and the formation of free radicals.
First, incident radiation by ionizing water molecules can produce an ion H2O+ (water cation) and a free electron, see the chemical reaction (1). This electron is called an anaqueous electron because it is very slow since almost all the energy has been invested in tearing it from the molecule. The ion H2O+ is very unstable and rapidly decomposes into an H+ (hydrogen cation) and a radical OH* (hydroxyl radical), see the chemical reaction (2).The aqueous electron can react with other organic molecules or with a second molecule of water producing H* radicals (hydrogen radical) and hydroxyl ions OH-, see the chemical reaction (3). The radicals H* and OH* are neutral molecules with great chemical reactivity since they have an unpaired electron that, with very little effort, will tend to create bonds and steal atoms from other molecules that, in the worst case, could be functional bio-molecules such like proteins or nucleotides.
The molecules H2O+ and H2O- are the cation and anion of water, respectively. Hydroxyl ions, OH-, and free protons, H+, being particles with opposite charges, are not dangerous because they attract and neutralize, forming new water molecules. Neutral radical molecules, H* and OH*, are dangerous because they will drift in the cellular environment until they affect a molecule of biological importance. The asterisk, *, in these and other radicals indicates that they are molecules that have a significant excess of energy. Furthermore, the radical OH* has a useful life of 10-9 seconds and, once formed, oxidizes almost instantly any molecule that is nearby 11.
The H* and OH* can recombine to form other free radicals or compounds with similar behavior, for example, recombination
forming hydrogen peroxide or oxygenated water, toxic at the cellular level; in addition to recombination
where the hydroperoxide radical is generated, an equally toxic compound that binds with another molecule of the same class can also form hydrogen peroxide.
Other free radicals can be formed, known as organic free radicals, which share with the precedents the fact that their excess energy can be transferred to the molecules, in particular to deoxyribonucleic acid (DNA), and break their chemical bonds. These organic free radicals are formed only when oxygen molecules are present 11.
There is also the possibility of forming free radicals with the alone excitation induced from the radiation on a water molecule, see the chemical reaction (6). The phenomena that occur when the water molecule is excited are not well known, but theoretically, its dissociation into H* and OH* radicals is possible. In one way or another, radicals are formed that do not have paired electrons, which makes them highly reactive, either as oxidizing or reducing agents.
Radicals are distributed heterogeneously along the path of the incident ionizing particles, depending on the linear energy transfer of said particles. A good part of them are lost in neutralizing reactions, combining as follows
In the case that free radicals have been generated in the cell nucleus, these propagate inside it, see chemical reactions (9) and (10), having the ability to attack DNA strands.
3.A geometric design of the physical system and simulation (TOPAS-nBio)
When ionizing radiation strikes human tissue, the greatest probability of interaction
is with water molecules, producing the so-called water radiolysis. Dissociative
processes such as H3O-+ OH*, (100% Probability), OH + H (65%
Probability), and H2O +
For this study, secondary radiation is used, that occurs in the platinum atom, specifically the Auger electrons generated by applying external radiation (electrons with an energy of 5 keV) to the physical system shown in Fig. 1. The generated Auger electrons conform an energy spectrum of the order of eV to some keV, with an average energy of approximately 1967 eV 12. The most energetic Auger electron emission comes from the innermost layers of platinum, which will strongly ionize the medium of interest; however, many others will have little influence on the interaction medium due to its low energy. The reactive oxygen species that will be produced in the medium of interest were simulated with Auger electrons with energies of 1955, 1957, 1960, 1967, and 1988 eV, of said spectrum.

Figure 1 The cisplatin molecule (green color) and the trajectories of the energy deposition of the Auger electrons (red color) are shown. Also the cell (blue color) where the reactive oxygen species are generated is indicated. (For clarity, the figure shows a single cisplatin molecule and the cell).
For the geometry of the simulation (using TOPAS-nBio code), a sphere with a diameter of 10 μm will be considered, and be filled with water, which will simulate a human cell (biological molecule). The isotropic source (Auger electrons from the platinum atom) will be located 3 nm from the cell surface, which 3 nm of the radio sphere that simulates being a cisplatin molecule. The dose deposited in the biological molecule is determined per emitted particle (Auger electron), understanding that not all particles will affect the cell. See Fig. 1 for more detail on the simulation geometry. The choice of liquid water is due to the lack of effective sections for specific biological materials in Geant4-DNA / TOPAS-nBio. Now, for the radiation source, it is considered (a) to simulate the indirect effect of cisplatin on the human cell, Auger electrons of 1,955 - 1,988 keV generated from the center of cisplatin with isotropic moment were considered; (b) monoenergetic spectra and a flat energy spectrum (same probability of occurrence) were considered for each Auger electron energy; (c) to avoid increasing the computing time, the direct interaction of the cisplatin molecule with external radiation was not simulated, it was considered that cisplatin is continuously emitting Auger electrons. Finally, for chemical considerations, the following was taken into account : (a) the effect of oxygen on the calculated G values was not considered; (b) neither was any chemical solvent taken into account, which can affect H* and OH* radicals, or any other molecule of water; (c) since we are interested in damage to the human cell, only free radicals were generated within the human cell, even though these are also generated outside of it.
The Auger electrons are generated from the center of an orb (cisplatin molecule) or a world of 3 nm of radio whose surface is in contact with the surface of another orb (the cell); all in a universe of 12 μm in diameter, large enough to contain both the cell and the cisplatin-source. Calculations were made to obtain the G value (number of reactive species per 100 eV of deposited energy) of reactive oxygen species produced inside the cell due to the interaction of electrons when traveling inside the biological molecule. It is relevant to note the differences in size between the two different orbs, which are shown in Fig. 1. Due to the independence of events (trajectories or histories of ionizing particles), with this simulation, a finite number of cisplatins can be placed (orbs) covering the biological molecule.
4.Results and discussion
The interaction of the Auger electrons with the cell, and in general with the matter, takes place through elastic and inelastic collisions. Following the trajectory of each Auger electron, its energy loss in the medium and the effects it causes can be quantified, in our case, the amount of ROS produced inside the aerobic cell (the spherical volume of water and whose diameter is 10 μm).
It is very interesting to visualize how the aerobic cell, in a natural way, generates the hydroxyl radical, which is lethal for cellular structures, for example, DNA and proteins. The oxygen molecule can be described as bi-radical since it has two unpaired electrons, each located in a different π* anti-bonding orbital. This is the most stable state of oxygen and is called the ground state. The oxygen in its ground state, despite being a powerful oxidant, is not very reactive. The reactivity that would be expected in the oxygen molecule in its ground state is reduced due to the parallel directions of the spins of its two unpaired electrons. If oxygen tries to oxidize another atom or non-radical molecule by accepting a pair of electrons, they must have parallel spins to engage in the vacant spaces of the π* orbitals. According to the Pauli exclusion principle, the spins of the electrons in an atomic or molecular orbital must have opposite directions. This fact, therefore, imposes a restriction on oxygen oxidation reactions. Although in principle, spin restriction seems advantageous for aerobic organisms, because it slows down oxygen reactions, it creates a situation in which the transfer of an electron can occur, thus allowing the formation of a free radical 13.
The reactivity of molecular oxygen can be increased by spin inversion (through an
energy impulse originated by the different biological pigments when they absorb
light of a certain wavelength) from one of the electrons of their external orbitals
to form the singlet oxygens, or for its sequential and univalent reduction to
produce oxygen free radical intermediates, see Figs.
2 and 3, respectively. Thus, giving
rise to the generation of oxygen species that occur in biological systems:
O2
- superoxide anion radical; HO2* hydroperoxyl radical;
H2O2 hydrogen peroxide; OH* hydroxyl radical;
RO- radical alkoxy; ROO* peroxy radical;
The generation of the hydroxyl radical occurs through the Haber-Weiss reaction (13), which results from the balance of two reactions (11) and (12), reaction (12) is the Fenton reaction, which requires a transition metal 11.
The hydroxyl radical is a highly reactive species that can react with any biological molecule at a rate of 107-1010mol/sec; their half-life and radius of action are therefore extremely short, 10-9 sec and 30 x 1010 m, respectively 12.
Returning to our simulations of reactive oxygen species through the TOPAS-nBio code, the following simulations of some of these reactive species will be discussed.
In Fig. 4, it is observed that at the beginning of the production of the hydrogen peroxide, H2O2, there is a small percentage of this species; however, when the production time is 104 ps (= 10 ns), its concentration rises considerably, above 80% of its saturation; this happens for the 5 different energies (1955, 1957, 1960, 1967, and 1988 eV) used in the simulation. From this time on, the production growth of this species remains constant. It is evident that in a relatively short interval (nanoseconds), it grows rapidly (from the point of view of chemical processes, which are from 10-6 up to a few seconds), in an exponential way.
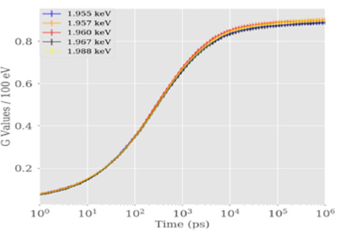
Figure 4 Generation of hydrogen peroxide (H2O2) as a function of the time and the energy of Auger electrons.
Now, in Fig. 5, it is shown the production of the hydroxyl radical, OH*, which has an inverse behavior to that of hydrogen peroxide. Where it is observed that its production decay of this radical is approximately 80% in a time of nanoseconds, which is consistent with its half-life 9,11. This last result shows that the hydroxyl radical “existence” time is around a few nanoseconds, which is in agreement with its half-life, as previously expressed. Since this radical is highly reactive and interacts with molecules “close” to it, including proteins or DNA. (It is important to mention that in all our results, the percentage statistical error remained below 5%, with a maximum of 4.37% obtained for the aqueous electrons).
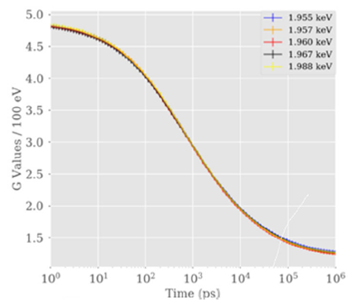
Figure 5 Production of oxygen species, especially the hydroxyl radical (OH*) as a function of the time and the energy of the Auger electrons.
Our interest in these two reactive species, H2O2 and OH*, is due to the fact that in our next work, cisplatin and some atoms of one species, transition metals, will be deposited on the surface of the SiO2 nanoparticles; when these two elements are internalized in the cell, to increase the number of OH* radicals, and to analyze a possible therapy against cancer through reactive oxygen species, in particular with OH* 7.
This kind of work has been studied before using mitochondria with the outer membrane coated in gold nanoparticles (GNP). In most cases 10 the goal was to identify dose enhancement effects, which correlates with direct DNA damage, but also the radiosensitization of cells, which cannot be explained with dose enhancements alone according to experimental results 14, such effects are often attributed to dose heterogeneity on length scales of hundreds of nanometers [hence in this work we show the possible effect on indirect DNA damage by analyzing the chemical output of the cisplatin molecule].
Our results are the premise to deeply understand the results when nanoparticles carry drugs to cancer tissues, and it is applied radiation (radiotherapy) 6. Regarding the area of the nanocomposites, it will be analyzed whether the radiation will increase or decrease the interaction between cisplatin and the surface of the nanoparticle and thus study the influence on the apoptosis process, in our next works.
5.Conclusions
We present new theoretical results through simulations carried out with the TOPAS-nBio code, which is an “efficient, effective, and versatile" version of Monte Carlo methods for numerical calculations in the deposition of energy in intracellular water. In the use of Monte Carlo simulations for the transport of radiation, we always seek to obtain a percentage of statistical uncertainty of less than 5%. This allows us to have a simple, clear, and correct interpretation of the underlying physics and biology in the generation of reactive oxygen species. The interaction of cisplatin and the surface of the nanoparticle was not considered in this work. However, there were results that coincide with the experimental ones, for example, the half-life of the hydroxyl radical.
It is important to analyze the generation of hydrogen peroxide (H2O2) and hydroxyl radical (OH*) because, in our next works, we will use transition metal ions (inside the cell) for Haber-Weiss and Fenton reactions (using H2O2). That results in resulting in an excess of hydroxyl radicals, which are lethal for proteins, lipids, and deoxyribonucleic acid. Also, it is interesting to note that this analysis is carried out on a simplified real physical system, and even so, some results agree with the experimental data 11.
It is suggestive to note that both reactive species, hydrogen peroxide and hydroxyl radical, seem to be linked, and, at the same time, to be complementary. They have a similar way of generation in the aerobic cell when they are naturally generated, as well as when they receive external radiation. This could lead us to think that there is an inherent and necessary mechanism for the existence of these two reactive oxygen species. Also it could indicate something about how reactive oxygen species behave in their generation and their interaction with organelles of the aerobic cell.
We have used the TOPAS-nBio code in cell biology, which is rarely used in this field. We researched to understand the underlying mechanisms of the action of Auger electrons to generate reactive oxygen species in cells, and this knowledge will be used in investigations of damage to cancer cells. The simulation of the physical and chemical aspects, in combination with representations of the extra / intra-cellular biological systems, obtained an adequate description of the deposition phenomena of the energy of the studied ionizing radiation and the subsequent phenomena, production of ROS, comparable with experimental biological results.