1. Introduction
The Gamow-Teller (GT) transition is without doubt one of spin-isosopin (στ) type’s most prominent nuclear weak transitions. They are not only involved in nuclear physics, they also play an important part in supernova explosions and nuclear synthesis. The GT reaction of core elements in the medium-mass region (MMR) is important to assess the pre-collapse production of supernova [1-4]. Electron capture reaction and β-decay were significant nuclear processes at the beginning of the supernova core collapse [5-7] Transitions of GT with ΔJ π = 1+ are mediated by a single στ operator and therefore do not have an orbital angular-momentum transfer (ΔL = 0) and spin-isospin flip-type (ΔS = 1 and ΔT = 1). Therefore, GT transitions are of type T z = ± 1, where T z is the third component of the isospin T which is given by T = (N - Z )/2 [8-10].
Saxena et al., conducted two ab initio approaches namely: The in-medium similarity renormalization group (IM-SRG) [19] and the coupled-cluster effective interaction (CCEI) to study of the strengths distributions of the transition strength of Gamow-Teller in some selected sd-shell nuclei. They also compared their theoretical results from the two mentioned approaches with the shell model calculations using the phenomenological USDB effective interaction [11]. Zegers et al., studied [12] the 24Mg(3He, t)24 Al reaction at E(3He)=420 MeV. An energy resolution of 35 keV was achieved. A recently developed empirical relation for proportionalities between Gamow-Teller and differential cross sections was used to extract Gamow-Teller strengths to discrete levels in 24Al. In the T = 1/2 mirror nuclei pair 23Na-23Mg. Fujita et al., studied the contribution of these different conditions, comparing the strengths of analog gamma M1 transitions and GT transitions deduced from high-resolution 23Na(3He, t)23 Mg charge-exchange measurements [13].
This study is aimed to calculate the GT strength distributions with higher energies of excitation. This could be very useful for future experimental data. The shell model calculations will be conducted using the shell model code NuShellX@MSU [14] to obtain the GT-strengths for 24Mg → 24Al, 24Mg → 24Na, 25Mg → 25Al, 26Mg → 26Na, and 26Mg → 26Al using USDA and USDB effective interactions in the full sd-model space. The results B(GT) values and their summed B(GT) will be compared with the corresponding experimental data.
2. Theoretical framework
The transitions are formed of two kinds under the selection rules for parity and angular momentum: GT transitions of Fermi (vector) and (axial-vector). Fermi transitions occur in a daughter nucleus only in isospin analog states where spin and parity are preserved (under conservation of isospin), i.e., |ΔJ = J i - J f | = 0, Δπ = π i π f = +1. The GT transition must satisfy the condition |ΔJ = J i - J f | = 0, ± 1, Δπ = π i π f = +1, (excluding 0+ → 0+). A shell model calculations without restriction were performed to describe the strengths distribution of the measured GT for sd-shell nuclei in the sd model space with USDA and USDB effective interactions [15]. The reduced GT transition strength B(GT) for the transition from the initial state with spin J i , isospin T i , and z-component of isospin T zi to the final state with J f , T f , and T zf is given by [16]
where τ ±1 = ± (1√2)(τ x + iτ y ) and a rank one tensor transform, and T z = (N - Z)/2. By employing the theorem of Wigner-Eckart in the space of the isospin, we get
where C GT is the isospin Clebsch-Gordan (CG) coefficient (T i T zi ± 1| T f T zf ) and the M GT (στ) is the GT matrix element of isovector spin-type.
From this expression for the “reduced” GT transition strength, we see that B(GT) consists matrix element of squared value of the isovector spin operator M GT (στ) and spin and isospin geometrical factors. Therefore, even if initial and final states are common, then the transitions are different B(GT) values in reversed directions. For example, the GT transition from a state having |JTT z 〉 of |0T 0 T 0〉 to the |1T 0 - 1T 0〉 state has three times larger B(GT) than that in the reverse direction.
3. Results and Discussion
3.1. 24Mg → 24Al
Figure 1 displays the calculated and measured strength distributions of B(GT) for the transition 24Mg → 24Al. The B(GT) values from ground state of 24Mg (0+) → 24Al(1+)states without any truncation using USDA and USDB interactions were calculated. The experimental data observed through the 24Mg(3He, t)24Al charge-exchange reaction observed at 420 MeV [17] and 24Mg(p,n)24Al reaction observed at 136 MeV [18]. Our results using USDA and USDB interactions agrees very well with the previous study conducted by [17] using USDA and USDB effective interactions. Also, our work agrees very well with the work of Saxena et al. [11] using different theoretical techniques. There are two dominant peaks at E x (24Al) = 0.889 MeV and E x (24Al) = 2.726 MeV with values 0.606 and 0.331, respectively, for USDA interaction. The two dominant peaks for USDB interaction. The two dominant peaks for USDB interaction located at E x (24Al) = 0.783 MeV and E x (24Al) = 2.805 MeV with values 0.537 and 0.441, respectively. Figure 2 represents the running sums of B(GT) versus excitation energy E x (24Al). The strongest peaks for the 24Mg(3He, t)24Al reactions observed at 1.090 MeV and 3.001 MeV with values 0.668 and 0.416, respectively. The strongest peaks for 24Mg(p, n)24Al reaction are found at 1.07 MeV and 2.98 MeV with values 0.613 and 0.362, respectively. The first and second strongest peaks calculated from USDA and USDB interactions comes from the transitions 24Mg (0+) → 24Al(1+ 2) and 24Mg (0+) → 24Al(13 +), respectively. The USDA and USDB interactions predicts correctly the ground state at 4+ which agrees with experimental observation. The accumulated sums of B(GT) given by USDA and USDB interactions are in better agreement with 24Mg(p, n)24Al reaction than 24Mg(3He, t)24Al reaction. The shell model resulting from both interactions is capable of explaining the observed GT transition strength concentrated at the energy of lowest excitation. Overall, the results of the shell model explained successfully the gross characteristics of the experimental B(GT) values as well as the summed B(GT) strengths.

Figure 1 Shows the theoretical values B(GT) in comparison to the corresponding experimental data [17,18] for 24Mg.
3.2. 24Mg →24Na
Figure 3 shows the shell model and GT strength experimental data for the 24Mg → 24Na transition. The B(GT) values were determined from ground state of 24Mg (0+ to 24Na (1+ states) without any truncation using USDA and USDB interactions. The are three experimental data available from 24Mg(3He, t)24Na [12], 24Mg(d,2 He)24 Na [17] and 24Mg(t, 3He)24Na [19] through the charge-exchange reaction the experimental data. The strongest peak in the observed experimental data for 24Mg(3He, t)24Na reaction is located at Ex(24Na) = 1.346 MeV with value 0.67 and the rest distribution of B(GT) lies in energy range Ex(24Na) = 3.14-3.94 MeV and Ex(24Na) =7.1 MeV. The strongest peak for the reaction 24Mg(t,3 He)24Na is found at Ex(24Na) =1.07 MeV with value 0.654 and the rest six values below 1 distributed in the range Ex(24Na) =0.44-6.87 MeV. The shell model calculations using USDA and USDB are quenched by a factor 0.59 to account for combination of configuration mixing with 2p-2h states as done in Ref. [12]. The strongest beaks found by USDA and USDB interactions are located at 5.067 MeV and 4.952 MeV with B(GT) values 0.564 and 0.37, respectively. These strong peaks predicted by USDA and USDB comes from the transition 24Mg (0+) → 24Na(19 +). The ground state of 24Na = 4+ is correctly predicted by both USDA and USDB interactions. Figure 4 displays the B(GT) running sums in terms of excitation energy of 24Na. The intensities calculated are similar to those measured for this transition. It is seen that the USDA and USDB interactions predicted the excitation energy agreed with the experimental data, the summed strength B(GT) as shown in Fig. 4 is closer to the experiment than the USDB interaction with the summed B(GT). The USDA and USDB accumulated sum of B(GT) strength calculations is in line with the experiment on higher excitation energy Ex(24Na) ≥ 5 MeV, but not on low excitation energy Ex(24Na) < 5 MeV, overall the summed B(GT) strength predicted by USDA interaction better than USDB matched with observed ones.

Figure 3 Shows the theoretical values of B(GT) compared to the corresponding experimental data [12,17,19] for 24Mg. The experimental data taken from.
3.3. 25Mg →25Al
Figure 5 displays the calculated and the measured B(GT) strength distributions for the transition 25Mg(5/2+) → 25Al(3/2+,5/2+,7/2+) without any truncation. The measured data observed through the reaction of charge-exchange 25Mg (3He, t) 25Al [22, 23]. The dominant B(GT) in the reaction value comes from the transition 25Mg([5/2]+ 1) → 25Al([5/2]+ 1), while the rest of B(GT) values are very low and not reliable [20]. The nuclei 25Mg and 25Al are very deformed nuclei and the energy levels for these mirror nuclei are very well described by using the particle rotor model [20]. Theoretical calculations using USDA interaction have three five strong peaks located at E x (25Al) = 0.0, 1.739, 6.213, 7.049 and 7.623 MeV, while the predicted USDB five strong peaks located at E x (24Al) = 0.0, 1.72, 6.346, 7.132 and 7.922 MeV. The ground state of both 25Mg and 25Al nuclei are correctly predicted as [5/2]+ by both USDA and USDB effective interactions. There are 40 calculated values of B(GT) comes from the transitions 25Mg([5/2]+) → 25Al(3/2+,5/2+,7/2+) 12 of them are zero, 5 are strong peaks and the rest 23 are very small values predicted by both USDA and USDB interactions. The calculation of the accumulated B(GT) strength values agreed very well with the data for 25Mg (3He, t) 25 Al [22] shown with green filled stripe, while the data taken from Ref. [23] with magenta color is not agreed with the theoretical predications of both USDA and USDB.
3.4. 26Mg →26Al
Figure 6 shows the strength distribution of B (G T) untruncated shell model calculations for the transition 26Mg (0+ → 26Al (1+) states using USDA and USDB interactions. The measured data obtained through the reaction of charge-exchange 26Mg (3He, t)26Al [23, 24] up to the excitation energy E x (24Al) 7.24 MeV. The experimental data for the reaction 26Mg (3He, t)26Al taken from Ref. [24] shown with filled square pints are shifted by 0.2 MeV on the x-axis to not coincide with the data taken from Ref. [23] marked with filled circulus. There are two strong peaks for the experimental B(GT) data from Ref. [23] located at 1.057 MeV and 1.85 MeV with values of 1.089 and 0.536, respectively, in the same manner, the data taken from Ref. [24] are very close in locations to the data of Ref. [23] which are located at 1.06 MeV and 1.85 MeV. Theoretical calculation with USDA predicts to strong peaks located at 0.0 MeV and 0.987 MeV, these strong peaks comes from the transition 26Mg (0+) → 26Al(1+)and 26Mg (0+) →26Al(1+), respectively. In the same manner, the USDB two strong peaks located at 0.0 MeV and 0.783 MeV, which comes from transition 26Mg (0+) → 26Al(1+ 1)and 26Mg (0+) → 26Al(1+ 2), respectively. The accumulated B(GT) strength values shown in Fig. 7 determined by using both USDA and USDB effective interactions agrees very well with the measured data and the USDB interaction accumulated values of B(GT) are more closer to the experiment than USDA
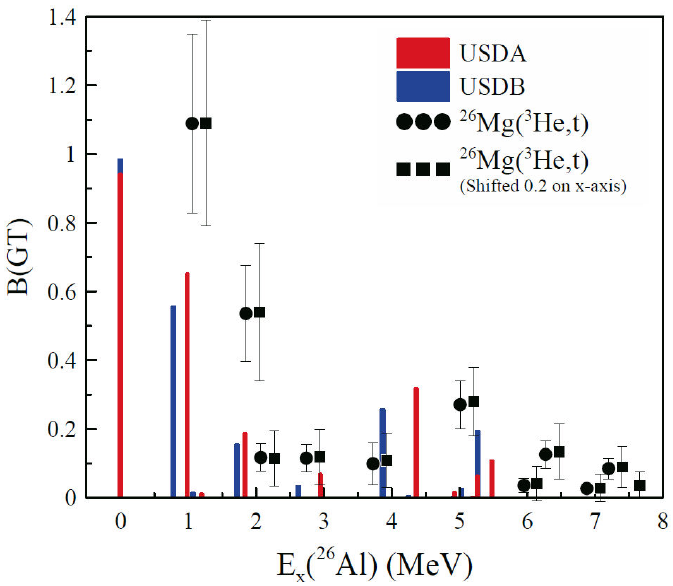
Figure 7 Shows the theoretical values of B(GT) compared to the corresponding experimental data [23, 24] for 26Mg.
3.5. 26Mg →26Na
Figure 9 shows the GT transition strengths for the transition 26Mg (0+) ground state to (1+) states. The experimental data observed from the reactions in 26Na is available from a 26Mg (t, 3He) experiment [23] and a 26Mg(d, 2He) experiment [24] presented in Fig. 9, and the calculation of the shell-models in full sd model space using the USDA and USDB interactions, respectively. The strongest peak in the 26Mg (t, 3He) reaction located at excitation energy E x (26Na) = 0.08 MeV and for 26Mg(d, 2He) reaction the strongest peak is located at also at E x (26Na) = 0.08 MeV. There are only four experimental values for both 26Mg(t, 3He) and 26Mg(d, 2He) reactions and they are distributed over excitation energy E x (26Na) = 0.08-5.02 MeV. Theoretical calculations of USDA and USDB interactions reach to exciation energy ∼ 12 MeV. The USDA and USDB predicted the strongest peaks at 11.939 MeV and 11.82 MeV, respectively. The strongest experimental peaks comes from the transition 26Mg (0+) → 26Na(1+ 1)for both 26Mg (t, 3He) and 26Mg (d,2He) reactions which disagree with theoretical predictions which comes from the transition 26Mg (0+) → 26Na(1+ 10) for both USDA and USDB, while the rest of transitions gives weak B(GT) strength distributions. The ground state spin and parity for both 26Mg and 26Na predicted correctly by both USDA and USDB interactions. Figure 10 displays the comparison of the accumulated sum of B(GT) values predicted by USDA and USDB along with the corresponding measured data. The USDA interaction are better than USDB interaction to describe the running sum of B(GT) values.

Figure 9 Shows the theoretical values of B(GT) compared to the corresponding experimental data [23, 24] for 26Mg.
4. Conclusions
In this work we report the result of the shell model in the sd model space for the recent measured data of GT strengths of 24Mg → 24Na, 24Mg → 24Al, 25Mg → 25Al, 26Mg → 26Na, and 26Mg → 26Al transitions. The results of both USDA and USDB interactions show reasonable agreement wit the available measured data. Our conducted study add more information on the GT strength distributions obtained in earlier work. For the individual B(GT) transitions, the qualitative agreement is obtained, while the predicted transition strengths sum is closely reproduced the observed data. This study might give useful information to researchers who are interested to study the B(GT) transition strengths in this mass region.