1. Introduction
In recent decades, quaternary Heusler alloys have attracted much interest due to their excellent properties such as high spin-polarization and half-metallic ferromagnetism (HMF), where they have possible uses in spintronic and thermoelectric applications [1-4]. The latest trend is towards looking for new sp or d 0 HM compounds, and some studies reveal that the materials without transition metal elements, d 0 compounds, would be a new type of HM materials where the spin polarization and magnetic order are mainly from the anion p-electrons of anions [5-7]. Many works on d 0 compound with ternary half-Heusler structure and full-Heusler structure have been done theoretically [8-12]. However, research and report on d 0 HM compounds with quaternary Heusler structure are still rare [13-17].
Compared with the traditional HMF quaternary Heusler with transition metal elements having large stray fields, these d 0 materials are more meaningful in real applications because of the smaller magnetic moments. Also the thermoelectric (TE) [1-4] quaternary Heusler materials are regarded as the alternate sources of energy due to their ability to convert waste heat into electricity and they have used for power generation because they are cheaper, abundant in nature, and environmentally friendly, i.e., free from toxic elements. To the best of our knowledge, no study on our d 0 compounds KSrCZ (Z = P, As, Sb) with quaternary Heusler structure has been reported in the literature. The characteristics of energy bands and the origin of the half-metallic gap were not studied. The effect of volumetric on the half-metallicity character was not investigated. The thermoelectric performances such as Seebeck coefficient, electrical and thermal conductivity, and thermopower factor are no calculated. In this paper, the structural, electronic, magnetic, and thermoelectric properties of new quaternary d 0 Heusler compounds KSrCZ (Z = P, As, Sb) have been studied by using the first-principles calculations. We have chosen this series of compounds with the hope that they would exhibit half-metallicity, good magnetic and thermoelectric properties. Our paper is organized as follows. The theoretical background is presented in Sec. 2. Results and discussion are presented in Sec. 3. A summary of the results is given in Sec. 4.
2.Computational method
The calculations were performed within the density functional theory (DFT) [18]. We use the full potential linear-augmented-plane waves the plus local orbital (FP-LAPW) method [19] implemented in the Wien2k package [20]. The exchange-correlation potential was treated under the generalized gradient approximation (GGA) [21]. The partial waves used inside the atomic spheres are expanded up to l max = 10 with a matrix size RmtKmax equal to 8. A 20 × 20 × 20 k-point mesh was used as a base for the in the first Brillouin zone was found to be sufficient in most cases. Within the (FP-LAPW) method, we impose a convergence criterion of 10-5 in the total energy to improve accuracy in the spin-polarized calculations.
3. Results and discussion
In general, the quaternary Heusler compounds are compounds adopted in LiMgPdSn-type crystal structure denoted as Y (space group 216) [22]. There are three possible different types of atom arrangement in the quaternary Heusler compound XX’YZ: Y1: X (0, 0, 0), X’ (0.25, 0.25, 0.25), Y (0.5, 0.5, 0.5), and Z (0.75, 0.75, 0.75); Y2: X (0, 0, 0), X’(0.5, 0.5, 0.5), Y (0.25, 0.25, 0.25), and Z (0.75, 0.75, 0.75); Y3: X (0.5, 0.5, 0.5), X’ (0, 0, 0), Y (0.25, 0.25, 02.5), and Z (0.75, 0.75, 0.75). To confirm the structural and magnetic ground states of Y1, Y2 and Y3 configuration, the total energies of the non-magnetic (NM) and ferromagnetic (FM) states as a function of the lattice constant of the three compounds were calculated and the obtained curves are shown in Fig. 1. The results show that the energies of FM - Y3 state are lower, indicating that FM - Y3 state is the ground state among three magnetic states. In Table I, we report our calculated equilibrium lattice constant a 0, along with the bulk modulus B 0, and the total energy Etot in their different structural and magnetic configurations. As shown in Table I, the obtained equilibrium lattice constant increases with the increase of the atomic radius of anion: 7.3218, 7.4775, and 7.8267 Å for KSrCP, KSrCAs, and KSrCSb, respectively. Also, the highest calculated bulk moduli for KSrCZ (Z =P, As, Sb) compounds in FM - Y3 configuration confirm the stability of this structure. Based on this, all the further calculations on electronic, magnetic, and thermoelectric properties of KSrCZ (Z = P, As, Sb) were performed on this structure only, i.e, in the type FM - Y3 structure.

Figure 1 Total energy as a function of volume per formula unit (f.u.) in the magnetic states FM - Y3 and NM - Y 3(a) and the three atomic arrangements type FM - Y1, FM - Y2 and FM - Y 3(b) for the KSrCZ (Z = P, As, Sb) compounds.
Table I Calculated total energies Etot (in Ry) per formula unit, equilibrium lattice constant a0 (in Å), the bulk modulus B (in GPa) for KSrCZ (Z= P, As, Sb) compounds in theirs different structures type and magnetic configurations.
Compound | structure | Etot(Ry) | a0 (Å) | B0 (GPa) | |
NM | FM | FM | FM | ||
KSrCP | Type − Y 1 | -8324.009601 | -8324.009398 | 7.0471 | 38.0318 |
Type − Y 2 | -8324.089225 | -8324.102824 | 7.3131 | 32.7388 | |
Type − Y 3 | -8324.098220 | -8324.112673 | 7.3218 | 32.5218 | |
KSrCAs | Type − Y 1 | -12162.030023 | -12162.029921 | 7.1744 | 35.6927 |
Type − Y 2 | -12162.098230 | -12162.099959 | 7.4516 | 29.8852 | |
Type − Y 3 | -12162.098405 | -12162.109866 | 7.4775 | 29.8643 | |
KSrCSb | Type − Y 1 | -20607.103600 | -20607.103042 | 7.4618 | 31.25 |
Type − Y 2 | -20607.153885 | -20607.16728 | 7.8367 | 28.1845 | |
Type − Y 3 | -20607.153835 | -20607.191886 | 7.8267 | 25.158 |
The calculated band structures of KSrCZ (Z =P, As, Sb) compounds with FM-Y3 type configuration at equilibrium lattice constants have been illustrated in Fig. 2. The spin-up triplet band crosses the Fermi level and exhibits a metallic behavior while the spin-down band are semiconducting with a forbidden indirect band gaps E g (W - Δ) around the Fermi level confirming that these compounds are half-metallic (HM) with 100% spin polarization. The predicted half-metallic gaps E HM are listed in Table II. This gap is known to be essential to describe the stability of HM magnetism of a half-metal [23]. As shown in Table II, KSrCP, KSrCAs, and KSrCSb display large HM gaps of 0.292, 0.234, and 0.351 eV, respectively, illustrating stable HM features. Unfortunately, no experimental measurements and theoretical data band gaps E g and E HM for the investigated compounds are carried for comparison.
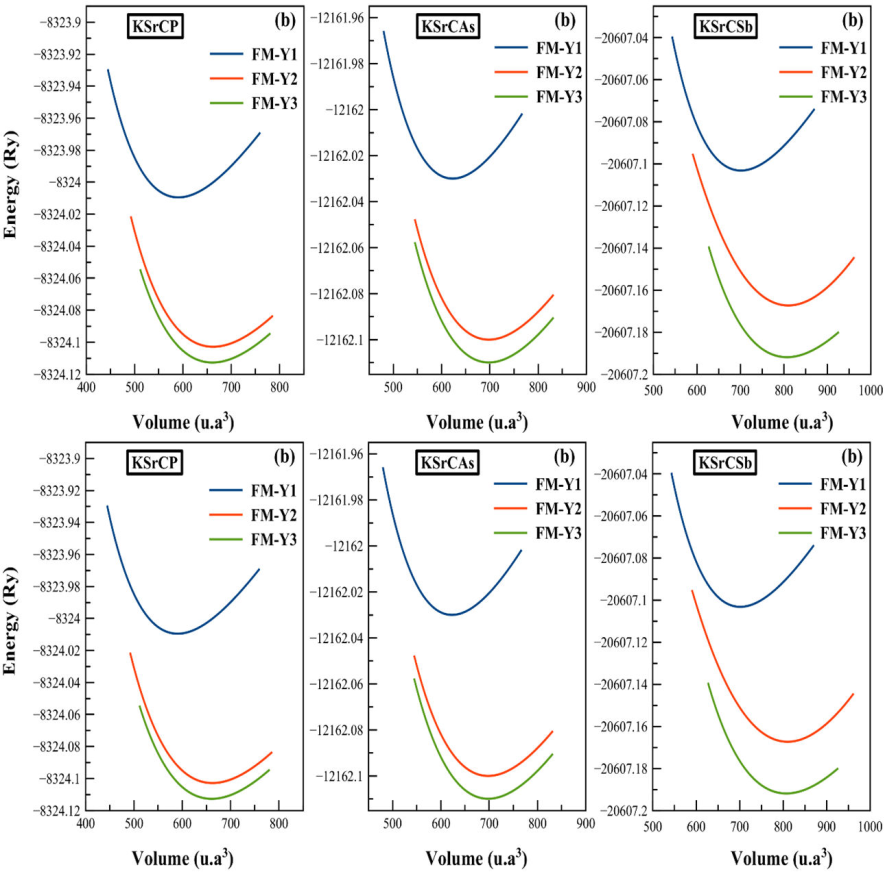
Figure 2 Spin-polarized band structure for the KSrCZ (Z = P, As, Sb) compounds at their equilibrium lattice constant.
Table II The semiconducting gap Eg (in eV), the half-metallic gap EHM (in eV), total magnetic moment μtot (in μB), the magnetic moment per atom (K, Sr, C, P, As, Sb), and magnetic moment in the interstitial region μint in compounds KSrCZ (Z= P, As, Sb).
Compound | Eg | EHM | µtot | µK | µSr | µC | µZ | µint |
KSrCP | 0.87 | 0.292 | 2 | 0.018 | 0.03 | 1.622 | -0.03 | 0.36 |
KSrCAs | 0.80 0.234 | 2 | 0.016 | 0.028 | 1.61 | -0.024 | 0.37 | |
KSrCSb | 0.92 | 0.351 | 2 | 0.01 | 0.02 | 1.618 | -0.028 | 0.38 |
To understand the electronic structure further, the calculated total and partial density of states (DOS) for these compounds are presented in Figs. 6-8. It can be seen that the DOS of all compounds have a similar structure. From partial DOS, it is clear that the spin-up triplet state across the Fermi level is mainly from C - p states with smaller contribution coming from the P -, As -, and Sb - p states. For the spin-down states of these materials, the top of the valence bands is mainly from the As - p states, while the bottom of the conduction bands is mainly from the C - p states responsible for gap formation. Our total magnetic moment μ tot per formula unit calculated is found to be integer values 2.00 μB for all compounds. This integer value is also a typical HM characteristic. We also list in Table II the local magnetic moments at the K, Sr, C, and Z (Z= P, As, Sb) sites. As seen in Table II, for all three half-metal compounds, the main contribution to the total magnetic moment is from p states of C.

Figure 6 The total magnetic moments for KSrCZ (Z = P, As, Sb) as a function of the lattice constants.

Figure 7 The variation of electrical conductivity σ/τ (a), thermal conductivity ·κ/τ (b) and Seebeck coefficient S (c) versus temperature in both the spin states for KSrCP.
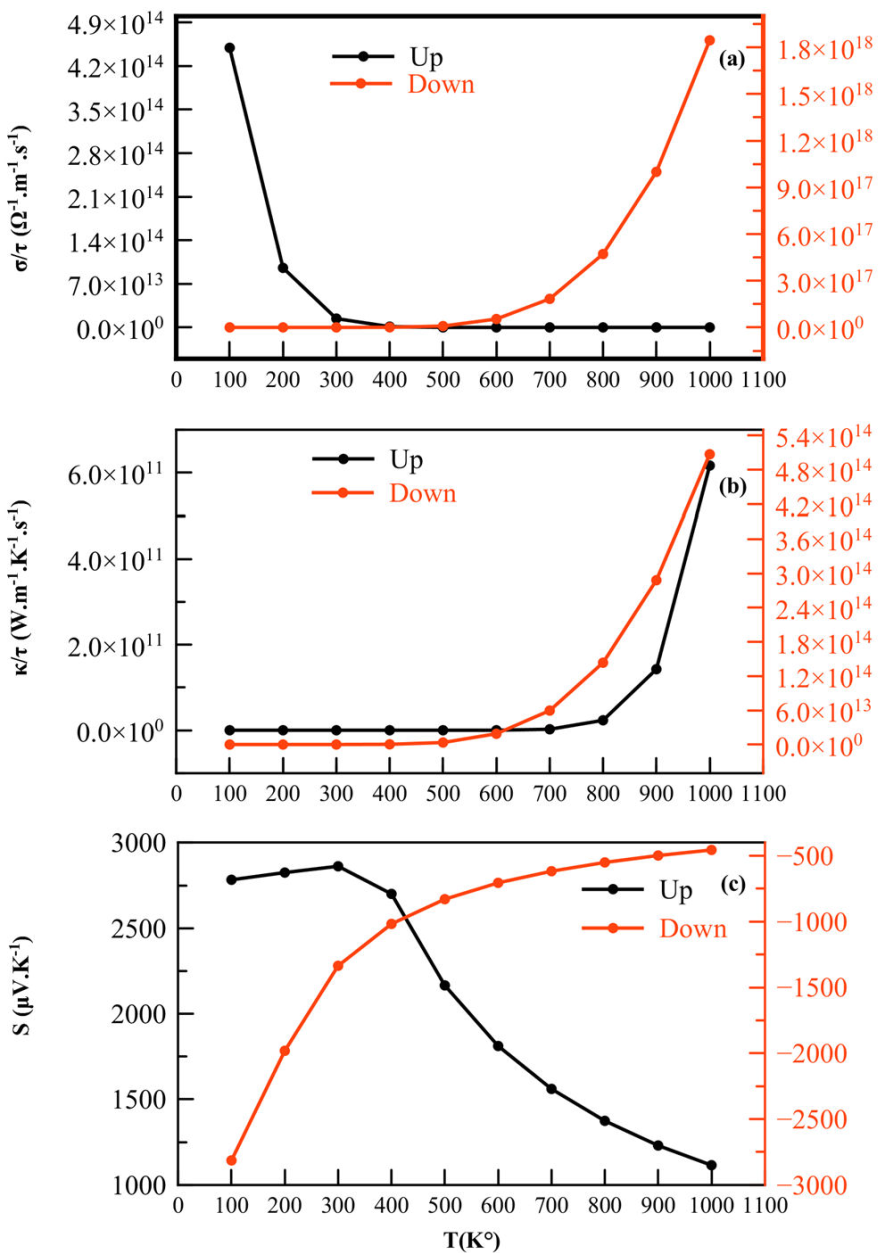
Figure 8 The variation of electrical conductivity σ/τ (a), thermal conductivity κ/τ (b) and Seebeck coefficient S (c) versus temperature in both the spin states for KSrCAs.
Because the half-metallic materials are usually prepared as thin films for spintronic applications, where the lattice constant of the half-metallic material strongly depends on the substrate lattice parameter and correspondingly the half-metallicity may be destroyed. Fig. 4 shows the total magnetic moments with respect to the different lattice constants. The magnetic moments remain integral 2 μB until the lattice is compressed to a critical value of 6.91, 7.17, and 7.38 Å for KSrCZ (Z = P, As, Sb), respectively. This indicates that the half-metallicity is preserved up to a lattice contraction of 4.18%, 4.17%, and 5.65% for all three quaternary Heusler compounds, reflecting the order of magnitude of half-metallic gap EHM. On the other hand, it is found that KSrCSb is the most stable compared to KSrCP and KSrCAs.
Thermoelectric (TE) materials transform the waste heat energy into usable electric energy, and thereby offer a possible solution to the present-day energy crisis. This category of materials is currently being investigated at faster rates than the other technologically important materials because of their eco-friendly and efficient energy management [24]. For the first time, the thermoelectric properties of KSrCZ (X = P, As, Sb) compounds are calculated by the BoltzTrap code [25].
In this study, we calculated the electrical conductivity σ/τ, thermal conductivity κ/τ, Seebeck coefficient S, and figure of merit ZT. An efficient thermoelectric material is required to have high electrical conductivity, low thermal conductivity, and a large Seebeck coefficient.
The temperature variation of electrical conductivity (σ/τ) is reported in Figs. 7-9(a). We observe that the electrical conductivity of three of these compounds decreases in the spin-up state, which confirms the metallic behavior in the spin-up state. While electrical conductivity increases with the increase in temperature, which again confirms the semiconducting behavior in the spin-down state and thus supports the band structure. The Electronic part of the thermal conductivity κ/τ shows a negligible variation up to 600 K and an exponential increase at higher temperatures, as depicted in Figs. 7-9(b). The Seebeck coefficients for KSrCZ (Z = P, As, Sb) compounds are displayed as a function of temperature in Figs. 7-9(c). The observed value of the Seebeck coefficient for all compounds are positive in the spin-up channel, signifying the presence of holes as charge carriers (p - type), while the in spin-down channel, the negative value of S suggests electrons as charge carriers (n - type).
The variation of the total electrical conductivity σ/τ as a function of the temperature is plotted in Fig. 10 (a). With the increase of temperature, σ/τ increases monotonously from a very small value until 500 K and beyond this temperature σ/τ increases linearly, and at 1000 K attains a value of 1.73 × 1018, 1.85 × 1018 and 1.21 × 1018 Ω-1 m-1 s-1 for KSrCP, KSrCAs, KSrCSb, respectively. This increase could be justified by the fact that increasing temperature enhances the thermal energy of the electrons to freely form a high conducting state.
Figure 10 (b) displays the temperature dependences of total thermal conductivity κ/τ. The κ/τ plots follow a similar trend as those of electrical conductivity σ/τ. The κ/τ value increases gradually, from nearly zero for all four materials in the range temperature between 100 K and 500 K. Beyond this temperature there is almost a linear increase in the range studied. The rise of the thermal conductivity with the increasing temperature is mainly due to the higher charge carrier concentration. Note in passing that the nearly indistinguishable plots (Fig. 2) suggest that KSrCZ (X = P, As, Sb) should have approximately the same band gaps (0.87, 0.8, 0.92 eV, respectively).

Figure 9 The variation of electrical conductivity σ/τ (a), thermal conductivity κ/τ (b) and Seebeck coefficient S (c) versus temperature plots in both the spin states for KSrCSb.

Figure 10 The variation of total electrical conductivity σ/τ (a), total thermal conductivity κ/τ (b), total Seebeck coefficient S (c) and figure of merit ZT (d) as a function of temperature for KSrCZ (Z = P, As, Sb).
We also computed the total Seebeck coefficient S variation calculated by a two-current model [26] to designate its nature, as shown in Fig. 10 (c). As one can see, the Seebeck coefficient of the all KSrCZ (X = P, As, Sb) Heusler compounds is negative for the entire temperature range and this negative sign of S explains that the electrons are dominant charge carriers, which in turn states that the spin-down state is dominant. Therefore, the KSrCZ (X = P, As, Sb) are n-type materials. The sharp increase in the S value at lower temperatures (< 200 k) indicates the presence of a low carrier concentration. In the range of temperature (200-1000 K), we see a slight linear decrease of the absolute value S, and at 1000 K attains a value of −461.41, −455.36 and −508.22 μV K-1 for KSrCP, KSrCAs, KSrCSb, respectively.
Finally, the calculated transport coefficients are now used to estimate the thermoelectric efficiency through the figure of merit ZT measurement given by the relation ZT = (S 2 στ/κ) and shown in Fig. 10 (b). The material is considered as a good element for thermoelectric devices if his ZT is about or greater than unity [27]. From Fig. 10 (d) the calculated value of ZT is negligible up to 100 K but, it abruptly increases to a maximum high value of 0.957, 0.96, 0.957 at 200 K for KSrCP, KSrCAs, KSrCSb, respectively corresponding to the maximum of the Seebeck coefficient S (see Fig. 10 (c)). High values of the figure of merit suggest the good thermoelectric performance of these quaternary Heusler compounds, indicating they could be promising materials for applications in thermoelectric generators. The value of electrical conductivity, thermal conductivity, Seebeck coefficient, and figure of merit ZT at room temperature are summarized in Table III. These materials show higher efficiency for thermoelectric relatively in comparison for the other quaternary Heusler compounds, including transition metals [1,28-30].
Table III Values of electrical conductivity σ/τ (in 1013Ω−1m−1s−1), thermal conductivity κ/τ (in 109 W m−1K−1s−1) and Seebeck coefficient S (in µV K−1), and figure of merit ZT at 300 K for KSrCZ (Z= P, As, Sb) compounds.
Compound | σ/τ | κ/τ | S | ZT |
KSrCP | 1.18 | 6.71 | -2007 | 0.96 |
KSrCAs | 1.12 | 6.51 | -1336 | 0.93 |
KSrCSb | 0.16 | 1.23 | -1567 | 0.95 |
4. Conclusion
In conclusion, the electronic structure, magnetic, and thermoelectric properties of the quaternary Heusler alloys KSrCZ (Z = P, As, Sb) have been calculated using the first principles full-potential linearized augmented plane waves method within the generalized gradient approximation (GGA). In all compounds, the stable type FM + Y3 configuration structure was energetically more favorable. At the equilibrium lattice constant, our GGA calculations show that all compounds KSrCZ (Z = P, As, Sb) are half-metallic ferromagnets (HMFs) with a large half-metallic gap E HM . The half-metallicity is found to be robust to the lattice compression. Further, the transport properties of the material reveal some fruitful results. These materials exhibit high values of Seebeck coefficient and figure of merit with the room temperature values. Since these materials offer high spin-polarization, robust half-metallicity and high Seebeck coefficient make it credible applicants for spintronic and thermoelectric applications. Most of the investigated properties are reported for the first time and provide the reference for future experimental work.