INTRODUCTION
Since it was sequenced for the first time in the genome of prokaryotic cells and the discovery of its role in adaptive immunity, CRISPR/Cas (clustered regularly interspaced short palindromic repeats-CRISPR associated protein) has drawn great interest due to its potential use as a genome editing tool1. The CRISPR/Cas system consists of a series of palindromic repeats (sequences of several nucleotides whose reading frame is the same in both the 5′ to 3′ and the 3′ to 5′ directions) separated by nonrepetitive sequences called spacers, to which cas genes (CRISPR-associated genes) are associated. These genes encode endonuclease enzymes capable of cutting DNA strands in a site-specific manner2.
The main purpose of this review is to describe relevant aspects of this fascinating subject to the clinically oriented scientific community. After briefly addressing the history of CRISPR/Cas, we will explain the molecular basis of its capability to recognize and cut specific DNA sites among the billions of base pairs contained in the human genome and the possibility of repairing single-nucleotide mutations in practically any desired gene. We will review scientific work supporting current clinical applications of CRISPR/Cas therapy and will conclude with the important bioethical considerations surrounding this subject.
GENE EDITING TOOLS PRECEDING CRISPR/Cas
Gene editing is a technique that incorporates site-specific modifications into the genome, making use of the cell's DNA repair mechanisms. Such modifications have the ultimate purpose of regulating the expression of a desired gene3. Before the discovery of the CRISPR/Cas system, zinc finger nucleases (ZFNs) and transcription activator-like effector nucleases (TALENs) were used to edit specific sites on the genome. During the 1990s, the ability of ZFNs to recognize a 3-base pair sequence in a DNA locus and make breaks in both DNA strands (double-stranded breaks or DSBs) was discovered. Later, arrays with more than one ZFN were developed with the capability to recognize longer DNA sites. Nevertheless, ZFNs were demonstrated to be relatively inefficient in locating target genes, had cell cycle-specific effects, and could recognize and edit sequences different from the intended genes (also called off-target effects)3,4.
The development of TALENs improved the efficiency, specificity, and safety of gene editing, as this tool had the capability to recognize even a single nucleotide in the desired genomic region, reducing the risk of inducing off-target effects5. However, its larger size and complex structure made its programming process more difficult, and when used in vivo, there was a higher probability of DNA recombination. These outcomes suggested the need to develop safer and more efficient gene editing tools3.
HISTORY OF CRISPR/Cas
In 1987, Ishino et al. first observed and described a series of short, repetitive, and palindromic DNA sequences within the genome of Escherichia coli while studying the isoenzyme conversion of alkaline phosphatase. However, the biological role of these sequences remained unknown until that time6. In the 1990s, Mojica et al. observed a motif of repetitive and spaced DNA sequences in the genome of Haloferax mediterranei while studying both the transcription of archaea at different salt concentrations and the seawater quality on the Mediterranean coast of Alicante, Spain; they called these sequences short regularly spaced repeats (SRSR)7,8. Later, the research group directed by Jansen et al.9 observed similar sequences within the genome of numerous prokaryotic species and called them spacers interspersed direct repeats (SPIDR). In 2002, both groups agreed to use the term CRISPR, which was immediately accepted and has been used ever since8-10.
Simultaneously, a group of genes adjacent to CRISPR loci were discovered; these genes encoded nucleases involved in the adaptive immunity process of prokaryotic organisms and were called cas (CRISPR-associated systems)9. In 2006, Makarova et al. described the role of CRISPR in the adaptive immunity of bacteria, which is present in 45% of these species; this topic will be discussed along with the mechanism of action in the next section11.
Cas9 protein belongs to the Class 2 system and is the most widely studied due to its simple but specific and versatile mechanism of action; it can be found in some bacteria, including Streptococcus pyogenes. Cas9 constantly scans the genome for phage genes with great speed and precision, protecting bacteria after the first viral exposure12. Initially, four types of Cas were isolated (Cas1-Cas4), and the number of cas genes gradually increased to 45, which created the need to classify existing CRISPR/Cas systems1,10. This classification was last modified in 2020 and consists of two classes: Class 1 subdivided into types I, III, and IV, and requires more than one Cas to carry out its function; and Class 2 which includes types II, V, and VI, requiring only one associated Cas2,10,13.
The first experimental study using CRISPR/Cas was carried out in 200714. A phage (spacer) sequence was inserted into the genome of S. thermophilus; as a result, the cell gained immune resistance and memory against the inserted phage as well as against other phages containing a similar sequence10.
J. Doudna and M. Charpentier are considered pioneers in using CRISPR/Cas as a genome editing tool. In 2012, they described the ability of CRISPR/Cas9 to induce DSBs in DNA and increased the system's precision by adding a single-guide RNA (sgRNA)15,16. This work opened the possibility of using CRISPR/Cas as a therapeutic tool for genetic diseases, and the enormous potential of their contributions was recognized with the Nobel Prize award in Chemistry in 2020.
In 2013, Zhang and colleagues first recognized the possibility of using CRISPR/Cas in eukaryotic organisms, including human embryonic kidney cells and stem cells. They engineered an sgRNA and introduced the use of a deactivated variant of Cas9 (nickase/nCas9) in an attempt to reduce off-target effects17.
Following their recognition as genome editing tools, CRISPR/Cas9 systems have been optimized to identify and induce DSBs in almost any sequence of the genome. Accomplishments such as reducing its size, increasing its specificity, and discovering new functions have been the key to many CRISPR/Cas applications in the study and treatment of human diseases. Moreover, CRISPR/Cas systems may be associated with other editing enzymes, enabling more precise gene editing systems2,10. The timeline of the most significant cornerstones in CRISPR/Cas research is depicted in figure 1.
CRISPR/Cas, THE PROKARYOTIC IMMUNE DEFENSE
The genome-specific site, in which the CRISPR/Cas9 system is located, is formed by an array containing repetitive palindromic sequences separated by non-repetitive sequences, called spacers. Alongside this array, are the genes encoding Cas enzymes, and genes encoding the trans-activating RNA (tracrRNA) which strand is complementary to the repetitive palindromic sequences (Fig. 2)12.
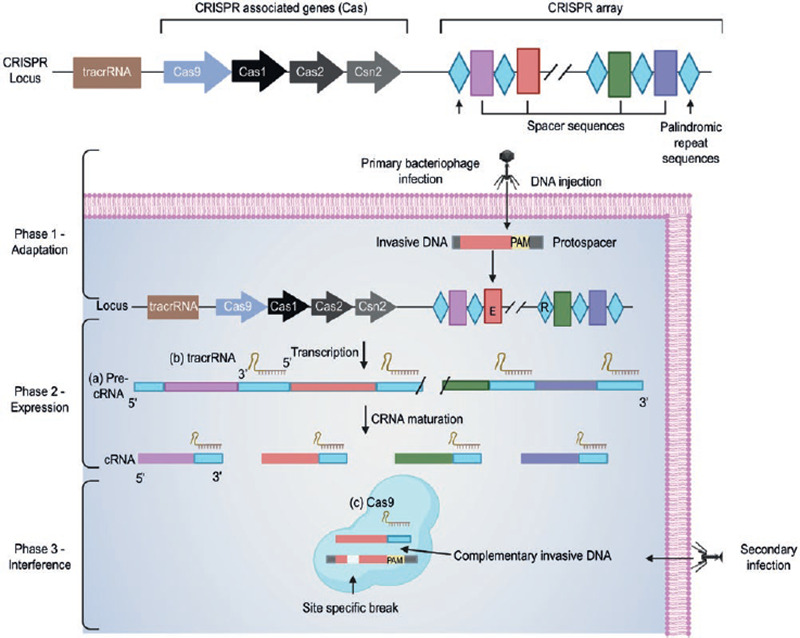
Figure 2. Class 2 CRISPR/Cas9 action mechanism in prokaryotic organisms (adapted from Jiang F, Doudna JA (2017)12, with permission from J. Doudna).Phase 1 - Adaptation: In this first phase, in response to an infection by an unknown bacteriophage or plasmid, the prokaryotic cell integrates a small sequence of the pathogen's genome into the CRISPR array as a new spacer through the acquisition machinery (Cas1, Cas2, Csn2)2,13. Phase 2 - Expression: This phase begins with the transcription of (A) a long precursor RNA (precrRNA) containing the repetitive sequences and spacers, (B) tracrRNA that has a complementary region to the repetitive sequences, and (C) a DNA endonuclease (enzymes capable of generating a double-strand DNA break) Cas9 encoded by the Cas genes. Once transcribed, the tracrRNA hybridizes with the precrRNA, and further cuts are made to form the mature crRNA-tracrRNA complex2,13. Phase 3 - Interference: In the last phase, the crRNA-tracrRNA complex merges with Cas9 endonuclease and directs it toward the target genome site that contains a complementary sequence. Once located, Cas9 cleaves the specific double-stranded DNA site. To perform this cut, CRISPR/Cas9 requires both the recognition of a specific region called PAM (which plays an essential role in the selection and degradation of DNA) flanking the target sequence, as well as the activation of the two Cas9 endonuclease domains. This results in the degradation of the foreign genetic material and the termination of a second infection by the same pathogen2,13,18.
As previously mentioned, CRISPR/Cas9 role in adaptive immunity was first discovered in bacteria and archaea. When a plasmid or bacteriophage infects the prokaryotic cell for the first time, small fragments of its foreign genetic material are integrated into the cell's genome. As a result, in response to a second infection, the cell will be able to recognize rapidly and destroy the pathogen. The CRISPR/Cas9 system immunity process includes three phases: adaptation, expression, and interference18. A simplified explanation of these three phases is presented in figure 2.
CRISPR/Cas9, THE MOLECULAR BASIS OF ITS CAPABILITY TO EDIT THE HUMAN GENOME
In contrast with CRISPR/Cas9 action mechanism in prokaryotic cells, human genome editing with CRISPR/Cas9 requires the creation of a sgRNA that mimics the tracrRNA-crRNA complex function. Therefore, sgRNA directs Cas9 endonuclease to the desired DNA target sequence to induce DSBs12. sgRNA is a chimeric RNA formed by two parts, a constant unit that allows Cas9 endonuclease binding and a complementary DNA sequence that binds to the target region in the genome2,19.
After DSBs are made, DNA is repaired by the host's cell-mediated mechanisms. The most common type is known as non-homologous end joining (NHEJ), characterized by random and error-prone DNA repairs (substitutions, insertions, and deletions with the development of frameshift, nonsense, and missense mutations), resulting in gene silencing or knockout (Fig. 3A)2,12,20.

Figure 3. Gene editing mechanisms using the CRISPR/Cas9 system.With the fusion of a Cas9 endonuclease and a sgRNA, the desired genome site is located and subsequently cut, generating DSBs. As a result, endogenous cellular mechanisms are activated, allowing DNA repair by (A) NHEJ, which causes random deletions, insertions, or substitutions that ultimately result in the silencing of a gene; or (B) HDR, which is a more specific pathway and allows the insertion of the desired gene sequence, with the disadvantage of requiring an endogenous or exogenous donor template, and this mechanism is only functional in specific phases of the cell cycle. Through the development of modified endonucleases that allow selective binding to the desired genome site without generating DSBs, (C) BE (ABE and CBE) can change a specific base for another allowing the conversion of all four types of nucleotides; and (D) prime editing replaces the sgRNA for a pegRNA that locates the desired sequence of the genome and contains the necessary information to encode the desired sequence using a reverse transcriptase12,20,23,24.
In contrast to NHEJ, homologous direct recombination (HDR) is another DNA repair mechanism that allows for desired gene replacement or knockin at a specific location within the genome, thus avoiding the introduction of random mutations. This mechanism requires an endogenous or exogenous template donor strand containing the sequence of interest12,20. However, the employment of HDR in CRISPR/Cas9-mediated gene editing is still limited, since this mechanism is only functional in specific phases of the cell cycle (Fig. 3B)21.
Treatment of monogenic disorders represents one of the most exciting research areas in the genomic and precision medicine field. Most of these disorders (58%) are caused by single nucleotide variants, including severe and prevalent diseases such as sickle-cell anemia, cystic fibrosis, and Duchenne/Becker muscular dystrophies2,21,22. In recent years, great progress towards the knowledge of the pathophysiology and molecular basis of these disorders has been made. However, current treatment options that impact patients' mortality and quality of life are limited. The development of novel treatment technologies such as the use of genome editing tools, including CRISPR/Cas9 system and related techniques (e.g., Base editors-CRISPR/Cas9 with other enzymes that are capable of inducing DNA modifications without generating DSBs), represents a promising and potentially effective treatment option in these patients.
Base editors (BE) represent a new gene-editing approach that combines CRISPR/Cas9 system with other enzymes that are capable of inducing DNA modifications without generating DSBs; instead, BE replace a desired nitrogenous base at a specific genome site, reducing off-target effects. This process requires nickase (nCas) and deactivated Cas9 (dCas9), which are generated by deactivating one or both Cas9 protein domains; these proteins maintain their ability to bind the target sequence but are unable to cut DNA and therefore, cannot generate DSBs20,23. There are two types of BE, cytosine BE and adenine BE. Both editors allow the conversion of all four types of nucleotides: C/T, A/G, T/C, and G/A (Fig. 3C)20,22.
In 2019, Anzalone et al. developed a new CRISPR/Cas9 technique variant known as prime editing. This variant principle combines an nCas9 with reverse transcriptase and an editing guide RNA (pegRNA), which contains the target sequence information and encodes the desired genetic information in a specific DNA site. Compared to other gene editing systems, prime editing has the potential to provide higher efficiency results with fewer off-target effects, since it does not require DSBs or DNA donor templates that other CRISPR/Cas9 editing techniques do (Fig. 3D)24.
CRISPR/Cas-BASED THERAPEUTIC APPLICATIONS IN CLINICAL MEDICINE
Hematological diseases: Thalassemias and hemoglobinopathies
Hematological diseases were among the first to benefit from the therapeutic applications of CRISPR/Cas9 technology (and its derivatives) due to the ease and effectiveness of extracting blood or bone marrow cells, modifying them ex vivo, and grafting them back into the host (ex vivo therapeutic strategy)25.
In 2020, a Phase II clinical trial proposed grafting CRISPR/Cas9-edited hematopoietic stem cells into the bone marrow of two patients with hemoglobinopathies for therapeutic purposes (Patient 1 with β-Thalassemia [β-T]; Patient 2 with sickle cell anemia [SCA])26. Both patients had life-threatening anemia refractory to conventional treatments; the biological therapeutic agent was called CTX001 (autologous PHSC CD34+ genetically edited with CRISPR/Cas9 to reactivate fetal hemoglobin production).
Patient 1 was a 19-year-old woman diagnosed with transfusion-dependent β-thalassemia (TDT). Given the severity of her condition, she required transfusion treatment from birth and started iron chelators at 2 years of age; before the clinical trial, the patient received 34 units of packed red blood cells (PRBCs) per year. Patient 2 was a 33-year-old woman with a complex genotype combining homozygous S alleles (bS/bS) plus a unique deletion in α-globin. Prior to the clinical trial, the patient had an average of 7 severe vessel-occlusive crises and 3.5 hospitalizations per year and needed 5 units of PRBCs per year.
The purpose in both cases was to increase fetal hemoglobin (HbF, α2γ2) transcription to compensate for the loss of function of the mutated or deficient hemoglobin.
In Patient 1 (TDT), HbF reached a proportion of 99% of total serum Hb, which increased from 9.0 g/dL to 14.1 g/dL at the end of the study. Total hemoglobin in Patient 2 (SCA) increased from 7.2 g/dL to 12.0 g/dL at the end of the study, of which HbF represented more than 40%; no off-target effects were observed. It should be noted that HbF was expressed in 98%-100% of circulating erythrocytes. These figures were maintained in Patient 1 and Patient 2 for 18 and 15 months of follow-up after the graft, respectively. In addition, the patients' symptoms subsided, and they stopped needing blood transfusions as part of their treatment regimen as soon as 30 days after the graft. The ex vivo therapeutic application of CRISPR/Cas9 technology completely eliminated the clinical manifestations in these two patients; this clinical trial exhibits promising results for the future treatment of severe and/or refractory hemoglobinopathies.
However, this therapy is not harmless. Both patients had severe adverse effects (AEs) throughout their follow-up, including pneumonia in the presence of neutropenia and hepatic a vein-occlusive disease with sinusoidal occlusion syndrome (VOD-SOS) in Patient 1, and sepsis in the presence of neutropenia, cholelithiasis, and abdominal pain in Patient 2. However, it is not clear whether the VOD-SOS in Patient 1 was caused by CTX001 directly or indirectly by performing a bone marrow graft in a patient with viral hepatitis. Similarly, several nonserious adverse events occurred multiple times over the study period, the most common being asymptomatic lymphopenia. Fortunately, all adverse events were resolved with medical treatment.
At present, at least 5 other clinical studies are being developed to expand ex vivo therapeutic applications of CRISPR/Cas9 technology in hemoglobinopathies26. These clinical trials are designed to integrate the findings of the experimental Phases I, II, and III of the use of CTX001 to treat 45 patients with TDT and 45 patients with SCA into a single project. Primary and secondary outcomes include several parameters that reflect the efficacy of CTX001 for each condition, as well as the presence and severity of AEs. The study is expected to be completed by October 2024.
In addition, an observational study was developed to follow the same cohort of 90 adult patients who received the biological agent CTX001 for 15 additional years (with an estimated term date in September 2034) to evaluate its safety and efficacy26. There is also a Phase III clinical trial designed to evaluate the safety and efficacy of CTX001 in 12 pediatric patients (2-11 years of age) with severe SCA refractory or intolerant to hydroxyurea. This trial is expected to be completed by May 202626. Furthermore, a single-arm, open, multicenter study is underway to evaluate the safety and efficacy of the biological agent ET-01, composed of autologous CD34+ human hematopoietic stem cells modified with CRISPR/Cas9, in patients with TDT26. This study includes eight participants (12-35 years old) and is estimated to be completed by mid-2024.
HEMATO-ONCOLOGICAL DISEASES AND MALIGNANT SOLID TUMORS
Another large group of diseases that have benefited from the use of CRISPR/Cas9 technology for therapeutic purposes are malignant neoplastic diseases. At present, this group of diseases has the highest number of in-progress clinical trials (Phases 1 and 2) due to their high individual and community impact, elevated morbidity and mortality, and disability-adjusted life years. One of the main strategies to treat these conditions using CRISPR/Cas9 technology consists of editing T-cells to increase their effectiveness in recognizing and attacking neoplastic tissue. To carry out this process, it is necessary to collect autologous T-cells for ex vivo genetic modification and then transfer them back to the patient to generate the expected attack against tumor antigens. Different genetic modifications can improve the effectiveness and aggressiveness of T-cells in defending the body against cancer.
Programmed cell death protein 1 (PD-1) is a receptor involved in a key immune checkpoint, as it acts as an immunosuppression mediator when activated by the ligand of PD ligand 1 (PD-L1); signals through the PD1 pathway contribute to the regulation of initial T-cell activation, fine-tuning of T-cells' fate and functions, and T-cell tolerance (by stimulating T-cell apoptosis)27. PD-L1 is expressed abundantly in the tumor microenvironment of various neoplasms and represents one of the main mechanisms that allow them to bypass the immune response of the host. Recently, the results of trials that explored blocking PD-1 and PD-L1 with monoclonal antibodies (pembrolizumab) have shown that blocking is clinically effective in helping treat patients with a variety of neoplasms susceptible to this intervention (including non-small cell lung cancer, NSCLC).
In 2020, Lu et al. published the findings of a Phase I clinical trial that sought to demonstrate the feasibility and safety of therapy with T-cells that were genetically engineered not to express PD-1 in 12 patients with refractory NSCLC28. As a secondary objective, they hoped to evaluate the efficiency with which edited T-cells could evade the tumor defense system (dependent on PD-L1) and, consequently, destroy malignant cells. In this trial, several Classes 1 and 2 AEs but no Class 3 AEs were reported; one of the patients did not experience any AEs. The AEs observed appeared to be dose-independent, and the most common were lymphopenia (n = 3), fatigue (n = 3), leucopenia (n = 2), fever (n = 2), arthralgia (n = 2), and exanthema (n = 2). No treatment-related deaths occurred. Regarding the effectiveness of the intervention, a mean disease-free survival of 7.7 weeks and a mean overall survival of 42.6 weeks were reported, and the disease control rate at 8 weeks was 16.7%. It should be noted that greater diversity in T-cell receptors (TCRs) in bone marrow precursor cells (BMPCs) has been associated with a better response to immune checkpoint inhibitors. In the study, a lower diversity in TCRs in significant BMPCs was detected using the Shannon diversity index (5.66 on average; compared with healthy donors, with an average of 8.11). One of the patients (a 55-year-old female with metastatic pulmonary adenocarcinoma with PD-L1 expression) maintained a stable disease for 76 weeks. Before infusion with edited T-cells, she had failed to gain any benefit from three different therapeutic regimens. A PET-CT scan performed 52 weeks post-infusion revealed that only one of the tumors showed an abnormal increase in metabolic rate. Biopsy analysis obtained 54 weeks post-infusion (compared with samples collected pre-infusion) showed minimal residual tumor tissue and prominent infiltrates composed of T-cells (CD3+/CD8+, the same phenotype as ex vivo edited T-cells) and macrophages.
Liu et al. used a different approach for editing T-cells: modified T-cells with a chimeric antigen receptor (chimeric antigen T-receptor, CAR-T)29. CART-cells are genetically engineered T-cells that produce an artificial T-cell receptor to provide a predesigned antigen recognition site for any desired protein, giving these cells a new ability to successfully target a specific protein or antigen, thus allowing their use in immunotherapy. This technique represents the main therapeutic approach for the treatment of hematological neoplasms, such as acute lymphoblastic leukemia, chronic lymphocytic leukemia, diffuse refractory large B-cell lymphoma, transformed follicular lymphoma, other non-Hodgkin lymphomas CD19+, and multiple myeloma, as well as for several highly relevant solid tumors, such as NSCLC, breast carcinoma, prostate carcinoma, glioblastoma multiforme, ovarian epithelial carcinoma, pancreatic carcinoma, and cholangiocarcinoma, among others.
Due to the high specificity and relative simplicity of using the CRISPR/Cas9 system, it is possible to edit multiple genes simultaneously. As a result, some approaches combine both strategies synergistically, generating CAR-Ts against solid tumors that express mesothelin but not PD-1, and for glioblastoma multiforme.
Moreover, Stadtmauer et al. edited T-cells to generate CAR T-cells (expressing the synthetic NY-ESO-1 TCR to recognize cancer cells with high specificity) and modified two other genes that favor the expression of this synthetic TCR over the wild-type TCR and simultaneously inactivate the PD-1 gene30. The modified lymphocytes were administered to three patients with refractory cancer (two with multiple myeloma and one with sarcoma). The results of this phase I clinical trial show that simultaneous editing of multiple genes with CRISPR/Cas9 in T-cells is effective and safe in patients with refractory cancer.
CARDIOVASCULAR DISEASES
Protein convertase subtilisin/kexin type 9 (PCSK9) is a protein produced in hepatocytes involved in the degradation of low-density lipoprotein (LDL) receptors. This function occurs both extracellularly, when PCSK9 binds to the receptor, and intracellularly, favoring the degradation of the receptor before its arrival at the cell membrane. Current strategies in the treatment of atherosclerotic arterial disease include the use of anti-PCSK9 monoclonal antibodies that exclusively inhibit the extracellular pathway and interference RNAs (iRNAs), such as inclisiran, that disrupt both pathways. A new alternative is the use of CRISPR/Cas9 to decrease the expression of the pcsk9 gene and thereby increase the high-affinity receptor-mediated uptake of LDL complexes from the systemic circulation by hepatocytes. In vivo trials in murine models have demonstrated the efficacy of this intervention to reduce pcsk9 expression to be as high as 90% and its efficacy to reduce circulating LDLs to be 40%31.
In a phase I study32, successful editing of the adult mouse pcsk9 gene occurred in 54-58% of hepatocytes, which resulted in a 44% reduction in circulating LDLs. Histopathological analysis of liver tissue did not reveal any toxic effects of the therapeutic complex. For this study, 10 mice, including 5 experimental and 5 control mice, were used. All mice were injected with a sgRNA for the Rosa26 gene as a marker to identify off-target mutations produced by Nme2Cas9 (Neisseria meningitidis 2 Cas9). The authors concluded that the application of Nme2Cas9, with a unique combination of characteristics (compact size, reduced PAM, extreme accuracy during mammalian editing, and unique AAV [adeno-associated virus] vector), promises to accelerate the development of genome editing tools for general and therapeutic applications with high potential for future human studies32.
Variants of this methodology, such as the AAV-CRISPR all-in-one self-cleavage system (which induces the destruction of the CRISPR/Cas9 complex after its action in the pcsk9 gene), have been reported to decrease the incidence of off-target mutations by as much as 20 times compared to previous reports without increasing toxicity or decreasing its effectiveness to inhibit PCSK9 and reduce circulating LDLs33.
NEUROLOGICAL DISEASES
Most cases of Alzheimer's disease (AD) are sporadic and multifactorial; the expression of the ApoE4 protein is associated with being a predisposing factor for AD development. CRISPR/Cas9 technology has been used in 3D organoids of human neural tissue to edit the ApoE4 allele, inducing it to express the ApoE3 protein instead, which has a normal function and does not favor AD onset. The ApoE4 allele is found in 40-50% of cases of early-onset AD and 80% of cases of late-onset AD34. The results suggest that the CRISPR/Cas-induced expression of ApoE3 can delay, prevent, or even reverse the neuron phenotype characteristic of AD. This conclusion is of substantial interest, as some studies suggest that the ApoE2 allele is a protective factor against AD. However, more evidence is needed to verify this assertion and to study the effects related to inducing its expression using CRISPR/Cas9 technology.
Another possible application of gene editing with CRISPR/Cas9 is amyotrophic lateral sclerosis (ALS). It is well known that 15% of the cases of this devastating disease are associated with genetic variants of the gene encoding the enzyme superoxide dismutase (SOD1); these mutations have autosomal dominant inheritance and high penetration. Several studies in murine models have shown that SOD1 editing is possible using the CRISPR/Cas9 system, resulting in a delay in the onset of ALS and thus increasing the life expectancy of animal models of this disease35.
Spinal muscle atrophy (SMA) is another disease that can potentially benefit from CRISPR/Cas9. In 2019, Valetdinova et al. analyzed the activity of CRISPR/Cas9 in exon 7 of the survival of motor neuron 2 (SMN2) gene in fibroblasts, induced pluripotent stem cells (iPSCs), and precursor cells of motor neurons (PMNs) obtained from a patient with SMA36. They identified that the use of single-stranded oligonucleotide donors ensures the efficient introduction of target T>C substitution in exon 7 of the SMN2 gene in iPSCs and PMNs, which is consistent with evidence that short oligonucleotide donors are preferable for recombination aimed at introducing nucleotide substitutions.
INFECTIOUS DISEASES
Human papillomavirus (HPV) is the causative agent of a sexually transmitted infection with the highest prevalence worldwide. The acquisition of HPV is associated to the development of carcinoma in infected tissues (mainly by high-risk serotypes)37. The efficacy of CRISPR/Cas9 technology to edit the E7 oncoprotein in HPV serotype 16 (HPV-16), has been demonstrated. In a study carried out in mouse models, nanoparticles with CRISPR/Cas9 were used that decreased the expression of the E7HPV16 protein in infected cervical cells. These findings suggest that the development of cancer cells and tumors can be decreased, and the malignant phenotype of the cervical epithelium may even be reversed37. Trials such as this represent new therapeutic (and even curative) applications of CRISPR/Cas9 technology against HPV infections in humans.
Coronavirus disease 2019 (COVID-19) has affected more than 500 million people all over the world, killing approximately 6 million people since the World Health Organization declared a pandemic status on March 11, 2020 (Coronavirus. July 04, 2022. Google News).
The phenomenon of “T-cell depletion” consists of the loss of the ability of T-cells to attack a virus or tumor when exposed to these pathogens (and their antigens) repeatedly, blocking the immune response that would allow their elimination38. It has been suggested that epigenetic changes are responsible for this process, making the host susceptible to disease again despite having previously generated an immune response. It is believed that in patients convalescing from COVID-19, this is one of the mechanisms that cause future reinfections, especially if the primary infection was mild (John Wherry, PhD, President, Department of Systems Pharmacology and Translational Therapeutics and Director, Penn Institute of Immunology, Perelman School of Medicine, University of Pennsylvania).
Of the different mechanisms proposed through which SARS-CoV-2 generates depletion of T-cells, two are of special relevance: (1) lymphocyte infection: while the virus cannot replicate inside the lymphocytes, it can access them, since they express a small amount of the SARS-CoV-2 receptor ACE2 (angiotensin-converting enzyme 2) on their membrane, stimulating apoptosis. (2) Induction of PD-1 expression: As a result of repetitive viral exposure, PD-1 expression is stimulated, facilitating lymphocyte apoptosis and decreasing its ability to identify and attack infection39.
A group of researchers have proposed to extract T-cells from patients with COVID-19 to genetically edit them ex vivo (using CRISPR/Cas9) to abolish the expression of PD-1 and ACE2 and subsequently generate an autologous transfusion of them (Phases I and II clinical trials) to evaluate the safety of the intervention (reported as toxicity or AEs associated with the drug), as well as its effectiveness to prevent reinfection by SARS-CoV-2 10 months after the last T-cells transfusion. Other variables recorded in the study are all-cause mortality and the magnitude of the immune response generated (reported through differential leukocyte count and quantification of pro-inflammatory cytokines). The estimated study population is 16 patients, and the expected completion date is November 2022.
Human immunodeficiency virus (HIV) is an infectious agent known to cause the acquired immunodeficiency syndrome (AIDS). The first experiment to genetically modify human embryos with CRISPR/Cas9 (causing germline cell mutations, affecting the embryo's entire genome and its offspring) was conducted in China in 2016 by Dr. He Jiankui, who edited (and hence inactivated) the CCR5 receptor in CD4+ T-cells of two twin human embryos of a couple with HIV infection (through deletion of a sequence of 32 amino acids, a mutation called CCR5Δ32) before grafting them to their hosts and continuing with the pregnancy40. In November 2018, Jiankui successfully announced the birth of the two twin sisters whose risk of HIV infection was considered to be minimal; it was also postulated that this immunity effect would protect them, even if they were exposed to the virus at any point in their lives40.
However, several studies suggest that the protection would not be complete, as CCR5 is only one of the potential pathways through which HIV can enter the cell. Moreover, CCR5 is not the most common cellular invasion pathway used by HIV in Chinese populations; CXCR4 represents an alternative pathway which remains completely viable. Furthermore, the role of CCR5 in the immune system is not yet fully understood, so it is not known whether the twin girls may be more susceptible to other infections throughout their lives.
As will be discussed later, the international recommendations for the correct use of CRISPR/Cas technology are firmly against its use in germline cells under any circumstances, particularly considering that in the case of the twins, there are other well-established and effective ways to both prevent HIV transmission and treat HIV infection.
Another strategy to prevent HIV infection through the use of the CRISPR/Cas9 system focused on editing the genes from the virus itself, mainly the long terminal repeats segments, whose interference generates excision of the HIV protovirus inside cells and key genes for the infectious process, such as gag, pol and env, among others. Although most of these studies have been performed in vivo in murine models and do not yet have human study counterparts, the successful excision and elimination of proviral DNA from HIV-1 by Cas9 (administered through AAV) lays the foundation for the design of clinical trials in humans. In 2014, a clinical trial was published in patients with HIV where autotransfusion of CD4+ T-cells modified ex vivo with ZFN to alter the CCR5 gene was performed; the results show that genetically modified lymphocytes are safe and effective for treating patients with HIV/AIDS41.
TYPE 1 DIABETES MELLITUS
Several studies with promising results have employed CRISPR/Cas9 technology for the treatment of type 1 diabetes mellitus (T1DM). It has been proposed to generate a reserve of pancreatic β-cells derived from iPSCs to be implanted when required (thus decreasing the rate of transplant rejection)42. iPSCs are a subtype of pluripotent stem cells derived from adult somatic cells that have been genetically reprogrammed to a state similar to embryonic stem cells through the forced expression of genes and important factors to maintain the characteristic properties of β-cells, particularly the capability to secrete insulin43.
An open-label, phase I clinical trial is ongoing to evaluate the efficacy and safety of an implantable VCTX210A device in 10 adults with T1DM for 6 months. The device is implantable, removable, and easily accessible, and contains pancreatic β-cells (PEC201A) that have been genetically engineered with CRISPR/Cas9 to evade the autoimmune response observed in T1DM, thus avoiding their destruction. Secondary outcomes are the host's immune response to the implant (innate and adaptive, including alloantibodies and autoantibodies) and the survival of the cells in the implant. This clinical trial is expected to end in December 2022.
MUSCULOSKELETAL DISEASES
To date, there is no effective or curative therapy for Duchenne muscular dystrophy (DMD); the only FDA-approved drug for this condition allows restoration of <1% of normal dystrophin protein levels44. Muscle stem cells (MuSCs) have great therapeutic potential for muscle genetic disorders. Zhu et al. conducted a study in which MuSCs were selectively cultivated from raw skeletal muscle cells of mdx mice, a mouse model of DMD45. By genome editing with CRISPR/Cas9, it was possible to correct the dystrophin mutation in cultured MuSCs, and after transplantation of these edited MuSCs in mdx mice, skeletal muscle dystrophin expression was restored. This represents a breakthrough in tissue stem cell-based therapies for DMD and other muscle disorders.
In another study, Min et al. demonstrated that it is possible to edit and correct the error in the dystrophin gene in vivo in murine models using an adeno-associated virus serotype 9, as well as ex vivo in iPSCs from human cardiomyocytes extracted from a patient with DMD44. This study described that the most common mutation (hot spot) in the dystrophin gene is located in exon 44; when present, this mutation disrupts the open reading frame of surrounding exons. Therefore, they proposed the use of a CRISPR sgRNA to knockout exon 44, allowing the transcription of dystrophin to proceed and restore protein translation and function. The preliminary results are promising; in the experimental group (murine models) that received a dose of AAV-Cas9 and AAV-sgRNA (at a ratio of 1:10), dystrophin expression strongly recovered, as evidenced by immunohistochemistry and Western blotting of rhabdomyocytes of the anterior tibial muscles, brachial triceps, diaphragm, and myocardium. In addition, dystrophin function was significantly restored and was considered similar to that in wild-type mice and much greater than dystrophin function in untreated DMD control mice (p < 0.001; n = 6). These findings suggest the possibility of correcting exon 44 deletions, either through the omission of the exon or the repackaging of surrounding exons, which is an important finding that could potentially be used to treat ~12% of patients with DMD44.
XENOTRANSPLANTATION
Organ transplantation is the ultimate therapeutic option for patients with organ failure syndromes of either congenital or chronic degenerative etiology. However, many factors, such as long waiting lists, elevated rejection rates, and post transplantation immune susceptibility, diminish the rate of successful outcomes of these procedures. In the last decade, efforts have been made to increase the number of successful transplants using xenotransplants (organ or tissue transplantation from a donor of another species than the recipient), as they represent a potential alternative to satisfy the demand for transplant organs. Nevertheless, organ rejection by the human recipient's immune system and the chance of disease transmission from the donor (zoonotic diseases) are the main concerns in xenotransplants. The Sus scrofa f. Domestica porcine species has become the most studied donor for organ xenotransplant to humans, as its anatomy and physiology are very similar and it has no direct phylogenetic association, representing a lower risk for zoonotic disease transmission46.
With CRISPR/Cas9 technology, numerous proposals aiming to reduce the rejection risk (genetically modified organs) have been made. In 2015, the group directed by Estrada successfully inactivated the main genes involved in acute rejection reactions mediated by the human immune system (ggta1, cmah, and β4galnt2) from the porcine genome, thus creating “triple knockout” donor models46.
Experiments in vitro involving two major gene modifications had a successful outcome in reducing transgenic organ rejection in human recipients. This was accomplished by inactivation of the genes responsible for eliciting cytotoxic reactions mediated by NK (natural killer) lymphocytes when exposing these cells to porcine antigens and insertion of the human genes encoding the CD55 and CD59 proteins46.
In 2021, two patients with declared brain death received the first genetically modified porcine kidneys; both were modified using CRISPR/Cas9. Following the procedure, there was no evidence of an acute rejection reaction or porcine endogenous retrovirus transmission; nonetheless, renal function remained diminished (the association between abnormal kidney function and the recipient's previous medical condition is unknown)47. Very recently, a patient diagnosed with congestive heart failure who did not meet the inclusion criteria for the heart transplant waiting list received the first porcine donor heart47. The procedure was approved by the FDA exclusively for this patient. This terminal patient survived for 2 months after the CRISPR xenotransplant, with no signs of organ rejection.
Advances in the field of xenotransplantation have led to the establishment of specialized centers with the common purpose of developing genetically modified organs and making them available for xenotransplantation. Two of these major centers are the University of Maryland Cardiac Xenotransplantation Program and the University of Alabama at Birmingham Comprehensive Transplant Institute; the latter is about to conduct a phase I clinical trial designed for 20 patients diagnosed with end-stage kidney failure who will receive genetically modified porcine kidneys. This study is about to begin the recruiting phase and is expected to last 6 years, as all 20 patients will be followed up for several years to evaluate the efficacy and safety of the planned procedure with particular attention to the immune response of the patients.
CRISPR/Cas: BIOETHICAL IMPLICATIONS
In the last decade, the applications of CRISPR/Cas9 system as a potential therapy for many diseases have rapidly increased because of its simplicity, relatively low cost, and high effectiveness. Therefore, this gene editing system has the potential to develop novel treatments for genetic diseases that otherwise could be lethal; to create resistance to certain pathologies or infections; be applied to other organisms to modify the biosphere for the human benefit; and to design almost any new experimental model that enhances our understanding of certain diseases and their pathophysiology.
In addition to its therapeutic potential in clinical medicine and as a research tool, a worrisome area in which gene editing could be used is for “eugenics purposes,” defined as gene modifications made to improve biological characteristics in the human species or the addition of new ones. However, establishing ethical guidelines in this subject is challenging, since it is not clear whether this type of practice should or should not be allowed. If permitted, what human traits might be improved? And, could those changes benefit an entire population and not just a small group of individuals? At this moment, international expert panels only recommend the use of CRISPR/Cas9 for therapeutic applications and discourage its use for eugenic purposes until ethical concerns are fully resolved48.
One of the most important ethical implications of CRISPR/Cas9 are the consequences of establishing desired gene changes in a target sequence and creating undesired changes elsewhere in the genome (off-target effects). Both could lead to the creation of new mutations that result in the formation of undesired products whose biological significance is unknown. Moreover, these harmful effects, if carried out in the germline cells, could be inheritable to the following human generations. Therefore, to enhance the safety of this genetic tool, the specificity of the systems must be improved, and the characterization and identification of the desired changes and off-target modifications in the genome should be made for further research. Another safety concern of gene editing with CRISPR/Cas9 is the creation of mosaicisms which occur when a modification is not carried out in all the cells of the individual. The cells that still have the mutation could cause the disease, making the editing process ineffective48.
In 2015, the First International Summit on Human Gene Editing was held, and the main purpose of this meeting was to develop recommendations for the proper use of CRISPR/Cas technology. Regarding basic and preclinical research, the expert panel emphasized the need to establish potential risks and benefits of gene editing in human somatic and germ cells. In the clinical field, they concluded that all CRISPR/Cas technology variants should only be used in somatic cells since germline cell editing would be irresponsible until higher levels of safety and efficacy are established. Finally, the Summit highlighted the need for creating worldwide guidelines to prevent any type of illegal or unethical activity arising from using CRISPR technology.
As was mentioned earlier in this review, in 2018, few days before the Second International Summit on Human Genome Editing (SISHGE)48 was carried out, a Chinese scientist, He Jiankui, announced the birth of twins whose embryonic genomes were edited using CRISPR (both of which are now known to be mosaics). Additionally, there was a third edited child whose medical follow-up was unknown. In response to this act of irresponsibility, the international scientific community exhibited complete disapproval and prompted an urgent call to stop the creation of edited embryos destined for implantation48.
It is worth mentioning that this act disregarded preexisting international agreements about CRISPR/Cas9 experimentation, which highly discouraged its use in human germline cells under any circumstances. In addition, most countries (including China, the United States, Mexico, and the European Union) have laws that support such agreements and criminalize germline cell editing. Dominic Wilkinson, a neonatologist, and professor of medical ethics at Oxford University, told The Lancet, “This was not a case of science outpacing ethical guidance or the law. There were guidelines in place that warned against the research of this sort. This appears to be a researcher who had no interest in attending to ethical guidelines relating to scientific research49.”
After Jiankui's announcement, the SISHGE established that despite the rapid progress in somatic cell line editing, its use in editing human germ cells destined for implantation purposes should not be allowed due to unknown risks derived from inducing genome modifications. However, it was also mentioned that in the future, germline cell editing could be approved as long as the existing risks were diminished and strict criteria were established50.
As previously mentioned, current guidelines state that CRISPR technology should not be used to perform genetic editing in germ cells or embryos whose purpose is implantation because of the unknown and serious potential consequences caused by off-target editing, the implications of editing the desired objective itself, the generation of mosaicisms (presence of two different cell lines originating from the same zygote), and the creation of new diseases and the risk of affecting an entire human generation with them50. Moreover, derived from the heritability of these edits, genetically edited individuals should maintain lifelong medical control so that if anomalies occur, they are detected and controlled48.
At present, no country has approved germline gene editing, and its application is either prohibited or poorly regulated. International consensus establishes that all nations must create practice guidelines, sanctions, and standardized policies prioritizing the safety of individuals whose genome is edited before allowing the clinical use of CRISPR/Cas9 technology. To this end, a safe transitional pathway should be created, including the necessary steps and recommendations to allow germline editing in human clinical trials50. These are the main guidelines proposed by The Royal Society in 2020:
- Editing should only be performed on embryos that will undoubtedly inherit a monogenic disease-causing severe morbidity and premature death, in some selected cases where some but not all embryos inherit the pathological genotype for severe monogenic diseases, and in cases where there are no other treatment options.
- The modification of the genome must replace the pathogenic variant with a known common sequence present in the general population.
- Gene editing should not be carried out on healthy embryos.
- Gene editing should not be used to improve human traits.
Furthermore, it is recommended that each country assemble an expert panel formed by the scientific community, experts in humanities, social sciences, ethics, and other relevant communities to analyze and debate other essential issues arising from the use of CRISPR technology50.
As a result of the rapid advances in the development of newer, more efficient CRISPR/Cas techniques and the perfection of the existing ones, it will not be long before human germ cell editing is permitted. In the perfect scenario, such approval could allow parents to have genetically compatible children who do not inherit a severe monogenic disease and have to deal with the associated symptoms and suffering48. However, to achieve this objective, the secure transition pathway guidelines must be strictly followed, prioritizing safety above research outcomes and personal interests to protect all individuals and future human generations.
CONCLUSIONS
CRISPR/Cas genes emerged in the evolution of prokaryotic microorganisms as an adaptative immune defense against viral infections. After the first exposure, viruses insert their genetic footprint into the CRISPR array contained in the bacterial genome. In the event of a second exposure to the same virus, the CRISPR genes and their associated nucleases specifically identify and cleave the foreign genetic material to destroy it, allowing survival of bacteria.
The most significant cornerstone in CRISPR research occurred almost simultaneously in the US west coast group led by Jennifer Doudna at Berkeley, and in the laboratory of Dr. Fen Zhang at Harvard University in Boston. Both research teams found that the CRISPR/Cas system could be used for gene editing in eukaryotic organisms. This discovery opened the possibility of editing almost any desired DNA sequence in animals and humans. This editing apparatus could potentially be trained to correct genes responsible for a myriad of inherited diseases in humans.
In the following years, great improvements were achieved in CRISPR/Cas technology, and new modalities of gene editing were designed to improve its efficacy and safety. Some of these techniques took advantage of the use of nickase (nCas) nucleases that maintained their ability to recognize specific DNA sites, but their capacity to cut DNA was nullified. These inactivated CRISPR/Cas nucleases can then be associated with other enzymes having the capability to edit nitrogenous bases without disrupting the double helix. Another technique uses reverse transcriptase and an editing guide RNA to repair DNA sequences without introducing frame shifts or other undesired mutations.
The possibility of using CRISPR/Cas for therapeutic purposes in humans is currently being evaluated in carefully designed trials worldwide. The list of diseases where CRISPR/Cas could be used is growing every day, including diverse clinical specialties such as hematology, oncology, neurology, cardio-metabolic, osteomuscular, and infectious diseases, among others. The possibility of editing genes responsible for the rejection of xenotransplants with CRISPR/Cas is an exciting research area that is currently being evaluated.
There is great hope that gene editing could offer a therapeutic alternative for many devastating health problems for which affected patients have no other option available. Nevertheless, editing the genomes of humans or any other species has to be seriously analyzed in terms of its bioethical implications. We strongly believe that CRISPR/Cas and other gene editing technologies have to be regulated by multidisciplinary teams of experts in international consensus panels. It is imperative to prohibit the use of gene editing for eugenics purposes under any circumstances.
At present, this technique should be restricted to somatic cell line editing, where it can offer a unique therapeutic alternative for life-threatening diseases. Editing germline cells poses even more difficult bioethical considerations, as the induced gene modifications could be inherited by subsequent generations. A pathway for proper use of gene editing in germ cells is being developed by international ad hoc committees, and these guidelines will have to be completely fulfilled and approved before any attempt to use them.
With all these important caveats in mind, we strongly believe that if properly used, CRISPR/Cas gene editing represents an extraordinary advance that allows clinicians to offer new hope to patients suffering from many devastating diseases.