1.Introduction
In the last decades, nanosatellites, also called CubeSats, have transformed the landscape of space missions, especially for Earth orbit exploration. Nanosatellites have also been widely used as technology demonstrators, scientific tools, and for educational purposes [1,2,3]. The design of the nanosatellite structures considers minimizing the weight without sacrificing performance because it is technically convenient to accommodate higher payloads and to maintain or even reduce the cost of the launch. The recommended structural materials for the development of CubeSats - [3,4] - include Al-7075-T73 and Al-6061-T6 alloys, due to their strength, weight, and coefficient of thermal expansion [5]. Alternatively, a few scientific groups have started to explore the use of composite materials for the fabrication of the panels constituting nanosatellites [2,6], an example being the SwissCube, launched in 2009 [7], that included carbon fiber-epoxy composite panels in its structural subsystem. Composite materials have a lower density than aluminum alloys and represent a viable candidate to replace aluminum in some of the structural subsystems of the nanosatellites if thermal property requirements are met [8,9]. According to Corpino et al. [4], one of the most critical issues for nanosatellite missions is the effect of the thermal environment on the nanosatellite material. Heat transfer phenomena (direct solar radiation, albedo, earth infrared radiation (IR), and internal heat generation due to on-board electronics) in space are mainly regulated by thermal conduction and radiation [4]. Therefore, one of the most challenging issues is for the material to manage temperature variations ranging from 100oC to - 130oC corresponding to exposure to direct sunlight and eclipse phases in extremely short periods [1]. The current thermal properties of polymeric matrices used in the manufacturing of composite materials need to be improved to comply with the required standard for the construction of CubeSats.
Polymer-based composite materials reinforced with ceramic filler powders offer a diversity of possible multifunctional applications [10,11]. Several functional properties such as electrical conductivity, piezoelectricity, thermal conductivity, and thermal stability can be improved, with the addition of reinforcing particles [12]. Epoxy resins, used as the matrix of carbon fiber composites for aerospace, aeronautical, and automotive applications [13], have been combined with a variety of fillers at high and relatively low particle loadings such as graphene (1, 3, and 5 wt. %) [14], graphene oxide at 0.5 wt. % [15], carbon nanotubes at 1 and 6.1 wt. % [16,17], barium titanate at 6 wt. % [18], alumina from 1 to 70 wt. % [19-22] or combinations of these nanoparticles [17,23] to improve fracture toughness, electrical properties, flame retardancy, UV-shielding [24], and wear resistance [20]. Few studies have focused on the study of thermal properties. Y. Feng et al. [25] proved that the incorporation of Al2O3 particles induces an enhancement of the thermal conductivity of epoxy with increments of 145, 200, and 317 % at 50, 60, and 70 wt. % filler loading, respectively. M. Wasim Akhtar et al. also reported an increase in the thermal conductivity of epoxy with increasing filler loading up to 50 wt. % [26]. Both studies concluded that high particle loading (up to 70 wt. %) must be employed to obtain an apparent functional property improvement, which in turn, increases the weight of the material, decreases the specific mechanical properties, and turns the polymer fragile [22].
To design ceramic reinforced composite materials for nanosatellite structures, it is
essential to improve the thermal properties (i.e., the thermal
diffusivity and conductivity) of the epoxy resins without considerably increasing
the weight of composite materials. The present investigation reports the effect of
micro- and nanoparticle low concentrations
2.Materials and methods
2.1.Particle preparation
The ZnO particles were prepared following the aqueous sol-gel synthesis method. Briefly, zinc acetate and oxalic acid were mixed at a molar concentration ratio of 0.1 /0.1 (M). The oxalic acid solution was added dropwise to the zinc acid solution, which was kept under constant agitation for 90 min (formation of the gel). The gel obtained was dried for 12 h at 60oC and then subjected to a thermal treatment at 600oC for 3 h.
The alumina particles were prepared by the citrate route. A polymeric citrate
precursor derived from a citric acid aluminum nitrate solution at a molar
concentration ratio of 0.5/0.1 (M) was used. The precursor thermal decomposition
at 60oC formed a precipitate that was dried at 200oC for 2
h, followed by thermal treatment at 900oC for 2 h. The synthesized
ZnO and Al2O3 particles were characterized in a SmartLab
XRD diffractometer from Rigaku in PB/PSA mode Parallel Beam/Parallel Slit
Analyzer with a grazing incidence angle (
2.2.Composite preparation
The resin compound Epolam 2500, a biphasic epoxy resin composed of primer and
hardener was purchased from Sika Advanced Resins
2.3.Thermal characterization
2.3.1.Modulated thermal response
Thermal images were acquired through an active infrared thermography setup,
consisting of a TTL controllable laser (120 mW LASER LDCU5, at 658 nm); a
function generator, for the generation of the TTL control signal; a closed
sample chamber; and an IR camera (FLIR, SC5000), suitable to operate in the
2.5 - 5.1
The capabilities of the IR camera electronics, along with its software, allow
the phase-sensitive images processing and synchronization to the reference
signal (from the function generator); so, there is no need for an external
lock-in amplifier module. The sample chamber was kept at high vacuum
(
2.3.2.Transient thermal response
The transient thermal response of each sample was sensed using a thermal
analyzer (Hot-Disk), which employs a very thin nickel wire (shaped like a
double spiral, encapsulated on a 25
2.3.3.Thermogravimetric analysis
The degradation kinetics of the composites were evaluated by
thermogravimetric analysis (TGA), performed in a PerkinElmer
3. Results and discussion
3.1.Ceramic-particles characterization
The acquired X-ray diffraction patterns of the
3.2.Thermophysical Properties
The slope method for thermal diffusivity measurements shows that for a homogenous thermally thin, sample the following relationship exists:
where

Figure 4 a) Δ ψ vs. r, and b)
Following the same data processing, the thermal diffusivity of the different
epoxy/

Figure 5 Obtained values for the effective thermal diffusivity, as a function of the particle content, for (a) Epoxy/Al2O3, and (b) Epoxy/ZnO composites.
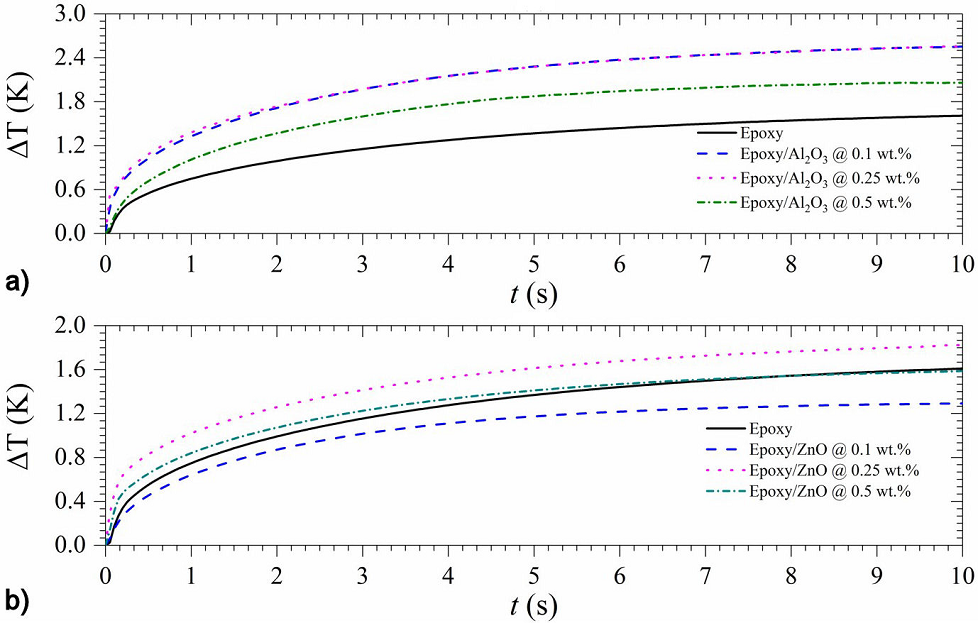
Figure 6 Thermal response (in amplitude) of a) Epoxy/Al2O3, and b) Epoxy/ZnO composites, recorded employing the Hot-Disk measurement system.
The effective thermal conductivity,

Figure 7 Effective thermal conductivity values of (a) Epoxy/Al2O3, and (b) Epoxy/ZnO composites, obtained from Hot-Disk measurement data.
A quite different thermal behavior occurs for the epoxy/ZnO composites, were a
non-monotonic dependency of
Considering that

Figure 8 Calculated C eff values of a) Epoxy/Al2O3, and b) Epoxy/ZnO composites, as a function of the particle content.
Again, the epoxy/
In Eq. (2), C(m), C(d) are the volume-specific heat capacity of the continuous
(neat epoxy matrix) and discrete phases (
3.3.Thermal stability and degradation kinetics
The thermal stability of the epoxy/

Figure 9 TGA thermograms for ceramic/epoxy and neat epoxy. The respective DTG thermograms for each concentration is displayed on the top right corner of the image. (a) Epoxy/Al2O3 and (b) Epoxy/ZnO.
The total mass loss for all samples was ranged from 79.8 to 76.9 %. The derivative of the thermogravimetric curves helps to determine the temperature at which the maximum rate of thermal decomposition is reached (see Fig. 9). DTG curves displayed in Figs. 9b and 9d show a three-stage degradation for the neat epoxy in a nitrogen atmosphere, which is affected by the increasing content of each oxide. The first degradation stage of the epoxy starts at 314oC and is associated with uncured epoxy resin and loss of volatiles. The second stage of degradation onset was detected between 318 and 330oC with a maxima temperature decomposition at about 340oC; this points out to the decomposition of aromatic functional groups. The last stage observed between 480 and 550oC corresponds to the breaking of the main chains of the epoxy resin. These polymer degradation stages match with data reported in the literature [15,38,39].
The DTG curves for all composites showed that the addition of ceramic particles
inhibited the first stage of the thermal degradation of epoxy, contributing to
the dissipation of its volatile fraction. These findings have not been reported
in other studies. The results demonstrate that for temperatures under
250oC, the addition of either ZnO or
The incorporation of
Thermal stability factors such as initial decomposition temperature (the
temperature of 5 wt. % loss,
Table I Thermal stability of epoxy/Al2O3 and epoxy/ZnO composites obtained from TGA thermograms.
Filler | Filler content (wt.%) |
Td5
(oC) |
Char at 900oC (%) |
---|---|---|---|
None | 0 | 288 | 23.08 |
0.1 | 288 | 20.20 | |
γ-Al2O3 | 0.25 | 288 | 20.20 |
0.5 | 273 | 21.34 | |
0.1 | 269 | 22.80 | |
ZnO | 0.25 | 269 | 22.80 |
0.5 | 269 | 22.80 |
The thermal properties in this study are compared for epoxy resin-based
composites produced by the above-mentioned process for particle loadings
Kinetic analysis of thermal degradation was carried out with Multilinear
Regression (MLR) method to fit four data curves obtained at a constant heating
rate by TGA in an inert atmosphere to the Arrhenius relationship,
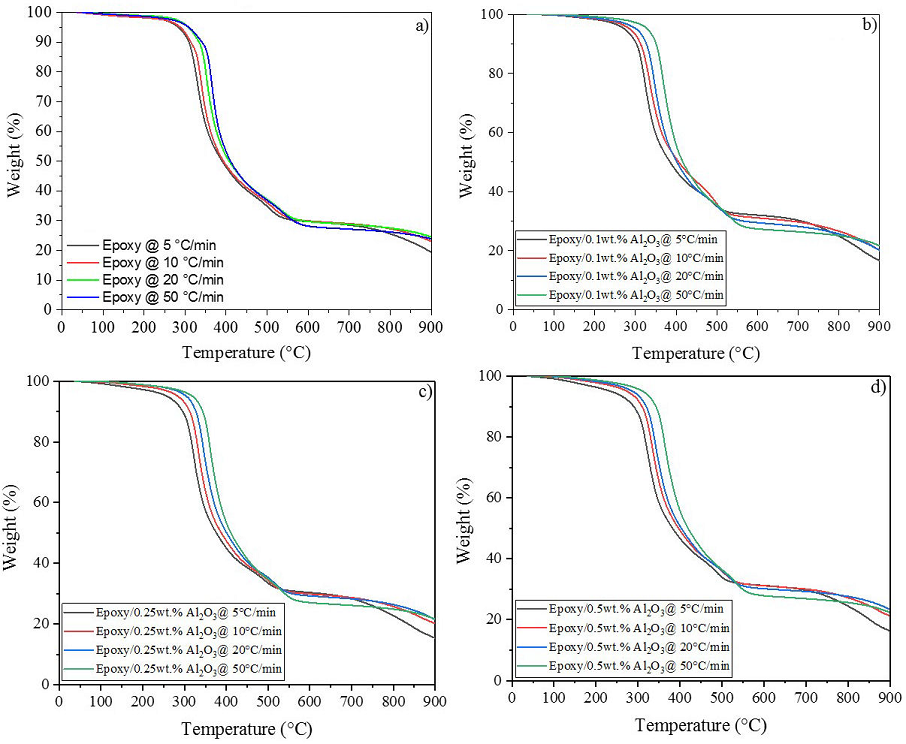
Figure 10 Thermograms of neat epoxy and Epoxy/Al2O3 at different heating ramps used to determine the kinetic degradation of the MRL method. a) Neat epoxy, b) 0.1 wt. %, c) 0.25 wt. %, and d) 0.5 wt. %.

Figure 11 Thermograms of neat epoxy and Epoxy/ZnO at different heating ramps used to determinate kinetic degradation of MRL method. a) Neat epoxy, b) 0.1 wt. %, c) 0.25 wt. %, and d) 0.5 wt. %.
Figure 12 presents the activation energy
(
4.Conclusions
The present study analyzes the effect of the incorporation of low contents (0.1,
0.25, and 0.5 wt. %) of citrate route
The inclusion of
The incorporation of
The results suggest that the incorporation of low loadings of alumina or oxide zinc nanoparticles increases the thermal properties and thermal stability of epoxy. These results also showed a feasible manufacturing method for lightweight epoxy-based composite materials that might be used for space applications (i.e. nanosatellites).