INTRODUCTION
Seagrass meadows play critical ecological roles in tropical and temperate coastal areas and are considered some of the most productive systems within marine environments (Phillips and McRoy 1980). In the Mediterranean Sea, Cymodocea nodosa and Posidonia oceanica form large meadows and are the dominant vegetation in coastal areas. In Mexico, Zostera marina is the principal aquatic vegetation that appears submerged in the coastal lagoons and shallow embayments of the Baja California peninsula and Gulf of California (Muñiz-Salazar et al. 2005). In addition to the importance of shoot and rhizome biomass, seagrass meadows serve as substrates for a large number of seaweeds, invertebrates, and vertebrates (Brito et al. 2005). As with other aquatic plants, seasonal fluctuations in seawater temperature and nutrient availability affect seagrass productivity and biomass and their associated epiphytes. For example, both the aboveground biomass and primary productivity of C. nodosa and Z. marina decrease in winter due to low seawater temperatures (Cabello-Pasini et al. 2004).
Along with biomass and primary productivity, biochemical components in seaweeds and seagrasses fluctuate in response to environmental conditions. For example, the chlorophyll content in the shoots of Z. marina and C. nodosa has been found to increase with depth and light limitation (Mazzella and Alberte 1986, Cabello-Pasini et al. 2003). Carbon and nitrogen content in the tissues of marine vegetation also vary based on fluctuations in irradiance, temperature, and the nitrogen concentration in seawater (Cabello-Pasini et al. 2004). Generally, the absorptance of seaweed tissues also varies based on chlorophyll and nitrogen concentrations and irradiance level. Thus, tissue absorptance fluctuates seasonally. However, the means and extent to which tissue absorptance and carbon and nitrogen assimilation are regulated by the nitrate concentration in seawater is not yet well understood in seagrasses, although it has been well established that water-column nitrate and reserve carbon levels activate nitrate reductase activity in Z. marina shoots (Touchette and Burkholder 2007).
Photosynthetic rates in plants are generally determined by evaluating oxygen evolution or carbon incorporation into tissues. Over the last 2 decades, fluorometry has proven to be a reliable and effective means to evaluate photosynthetic processes in marine macrophytes (Edwards and Baker 1993; Beer et al. 1998, 2000). Indeed, fluorometric methods that estimate photosynthetic characteristics are nonintrusive and much faster than oxygen evolution or carbon incorporation techniques. In particular, pulse amplitude modulated (PAM) fluorometry of in vivo chlorophyll fluorescence associated with photosystem II (PSII) can be used to monitor the primary reactions of photosynthesis under artificial or natural light conditions (Beer et al. 2000). Empirical studies have shown that the electron transport rate (ETR) is closely correlated with oxygen evolution and CO2 fixation in C4 plants (Edwards and Baker 1993), and values close to the theoretical ETR:oxygen production ratio of 5-7 mol electrons per mol of oxygen production have been reported in 2 Ulva species (Figueroa et al. 2003).
As in plants, photosynthetic rates in seagrasses depend on environmental factors, such as seawater temperature and irradiance, and biological factors such as the tissue type and long-term light-history of the sample. Both irradiance and seawater temperature regulate the photosynthetic rates of seaweeds and seagrasses. However, the effects of the nutrient concentrations in seawater on the ETR in these aquatic plants are less understood. Knowledge of the effects of nutrients on the ETR in the photosynthetic apparatus is critical for understanding the seasonal fluctuations of photosynthesis, growth, and other metabolic characteristics in seagrasses. In phytoplankton and macroalgae, optimum quantum yield has been shown to covary with the concentrations of silicon and phosphorus in seawater (Kolber et al. 1990, Young and Beardall 2003). Furthermore, it has been demonstrated that the nitrogen concentration in seawater regulates the relationship between ETR and gross photosynthesis in the green alga U. rigida (Cabello-Pasini and Figueroa 2005). Therefore, the aim of this study was to evaluate the effects of irradiance levels and nitrate concentrations in seawater on the ETR of the seagrass C. nodosa.
MATERIALS AND METHODS
Plant material
Cymodocea nodosa was collected in the intertidal zone of Fuengirola, Spain (36°30′20″ N, 4°39′10″ E). The samples were placed in ice coolers and transported to the laboratory within 2 h of collection. The effects of the nitrogen concentration in seawater on the reflectance, transmittance, effective quantum yield, and concentrations of nitrogen and carbon in the tissues were evaluated by incubating C. nodosa shoots in media with different nitrate concentrations. The shoots were incubated for 3 weeks in acrylic containers (2 L, 15 samples per container) with media containing 10 µM PO4 and 0, 2, 5, 25, 50, and 100 µM NO3 -. The nitrogen concentration in the media was less than 1 µM NO3 - and NH3 +. The media in the incubation chamber was changed every other day, and nutrients (KNO3, KH2PO4) were added from a stock solution. Samples were incubated at 15 °C and 100 µmol photon·m-2·s-1 using daylight fluorescent lamps (FL 18W; Osram, Munich, Germany). Shoot rhizomes were secured to the bottom of each acrylic container using a weighted plastic mesh. Filtered air was bubbled into each container to maintain the media in constant movement.
CHN Determination
After the incubation period, the samples were dried (60 °C) for 48 h. The content of intracellular nitrogen and carbon (% dry weight [DW]) was evaluated (n = 6) using a 2400 CHN elemental analyzer (Perkin-Elmer, Waltham, MA, USA).
In vivo chlorophyll a fluorescence
The photosynthetic rates of the shoots (n = 6) were estimated by measuring in vivo chlorophyll a fluorescence of PSII with a portable DIVING-PAM fluorometer (Heinz Walz GmbH, Effeltrich, Germany). Basal fluorescence (Fo) was estimated before sunrise after the natural darkness period (night). Maximum fluorescence (Fm) was determined in dark-acclimated shoots by applying a saturating actinic light pulse (9,000 µmol photon·m-2·s-1, 800 ms). Variable fluorescence (Fv) was calculated as the difference between Fm and Fo, and optimum quantum yield (Fv/Fm) was estimated as the ratio of Fv to Fm (Schreiber et al. 1994). The effective quantum yield of PSII (ΦPSII) was estimated in light-acclimated tissue according to the equation of Schreiber and Neubauer (1990):
where Ft is the intrinsic steady state fluorescence in the tissue under light conditions and Fʹm is the maximal fluorescence of light-acclimated tissue induced by a saturating actinic light pulse (9,000 µmol photon·m-2·s-1, 800 m s). The fiber optic component of the DIVING-PAM fluorometer was kept at a 45° angle from the plant. ΦPSII was determined throughout the day under natural solar irradiation.
The ETR through PSII was determined with the following equation:
where AQλ is the quantity of absorbed photons estimated as the product of the integration of spectral absorptance (Aλ) in the photosynthetic active radiation spectra (λ = 400-700 nm), and FII is the amount of AQ directed to PSII. The value of FII for Chlorophyta and seagrasses is approximately 0.5 (Figueroa et al. 2003, Johnsen and Sakshaug 2007).
Tissue absorptance (Aλ, n ≥ 6) was determined at 1-nm intervals between 400 and 700 nm using an integrating sphere (LICOR-1802) connected to an 1800 UW spectroradiometer (LICOR, Lincoln, NE, USA) according to the equation of Figueroa et al. (2003):
where Tλ is transmittance, and Rλ is the reflectance of the tissue.
Experimental design
Diurnal fluctuations in effective quantum yield were monitored in C. nodosa shoots that had been pre-incubated for 2 weeks at 25 µM NO3 - as described above. The shoots (n = 10) were incubated under different levels of natural irradiance in 30-L Styrofoam containers on the rooftop of a building. Natural incident irradiance (Eo) was reduced to 20%, 33%, 60%, and 100% Eo using screen mesh. Quantum yield measurements (n = 10) were conducted approximately every 2 h from sunrise to approximately 2 h after sundown. Incident solar photosynthetically active radiation (PAR) at the sampling site was measured every minute throughout the day using an ELDONET radiometer (Real Time Computer, Möhrendorf, Germany). The daily-integrated irradiance of quantum solar energy was determined from dawn to sunset by integrating the instantaneous irradiance values.
The effect of nitrate on the effective quantum yield of C. nodosa was evaluated by incubating shoots for 2 weeks at 0, 5, 25, and 100 µM NO3 - in acrylic containers at 15 °C and 100 µmol photon·m-2·s-1 using daylight fluorescent lamps (FL 18W, Osram) as described above. Shoots were transferred to 30-L Styrofoam containers under natural irradiance, which was attenuated to 33% Eo using mesh screens. Quantum yield measurements (n = 10) were conducted approximately every 2 h from sunrise to approximately 2 h after sundown.
Data Analysis
The effects of nitrate or irradiance on the effective quantum yield of C. nodosa were calculated using a one-way ANOVA. Homoscedasticity and normality of the data were evaluated using Bartlett’s and Shapiro-Wilk tests, respectively, and multiple comparisons were evaluated through Tukey post-hoc tests (Sokal and Rohlf 1995) in SigmaStat (Systat Software, San Jose, CA, USA). The significance of the Pearson product-moment correlations between effective carbon, nitrogen, the C:N ratio, effective quantum yield, and nitrate concentration in the media was evaluated at P < 0.05.
RESULTS
The carbon and nitrogen concentrations in the tissues of C. nodosa varied in relation to the nitrate concentration in the media (Fig. 1). An increase of 6.5% in tissue carbon levels (P < 0.05) was detected as the nitrate concentration in the media increased from 0 to 100 µM NO3 -. In contrast to the carbon concentration in the tissues, the nitrogen levels increased (P < 0.05) more than 60.0% in C. nodosa as the nitrate concentration in the media increased from 0 to 100 µM NO3 -. The tissue C:N ratio significantly decreased from 18.5 ± 2.2 in tissues incubated with no nitrate (0 µM NO3 -) to 12.6 ± 1.9 in tissues incubated with 100 µM NO3 -.

Figure 1 Carbon (C) concentration (% dry weight [DW]), nitrogen (N) concentration (%DW), and the C:N ratio in Cymodocea nodosa tissues incubated with different nitrate (NO3 -) concentrations. Data points represent the average of 6 samples ± SD.
The transmittance and reflectance of C. nodosa shoots were regulated by the nitrate concentration in the media (Fig. 2). Transmittance in the shoots exponentially decreased (P < 0.05) from 0.290 ± 0.07 in shoots incubated with no nitrate (0 µM NO3 -) to 0.198 ± 0.07 in shoots incubated with 100 µM NO3 -. Reflectance did not show a clear pattern with regard to the nitrate concentration in the media, although maximum reflectance values were observed in shoots incubated with 25 and 50 µM NO3 -.
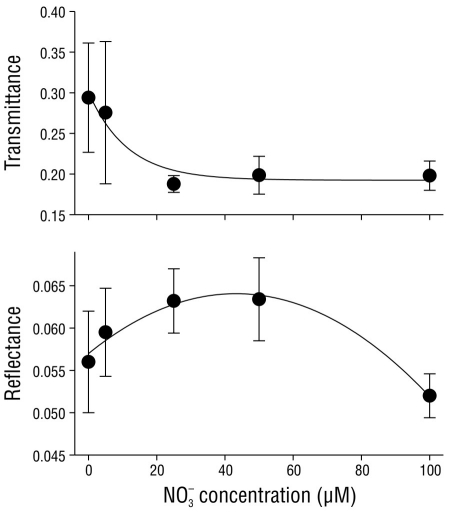
Figure 2 Transmittance, reflectance, and absorptance of Cymodocea nodosa tissues incubated with different nitrate (NO3 -) concentrations. Data points represent the average of 10 samples ± SD.
The effective quantum yield in the shoots of C. nodosa was affected by the nitrate concentration in the media (Fig. 3). The ΦPSII values significantly increased (P < 0.05) from 0.70 ± 0.03 in shoots incubated with no nitrate (0 µM NO3 -) to saturation (0.78 ± 0.01) in shoots incubated with 50 and 100 µM NO3 -.
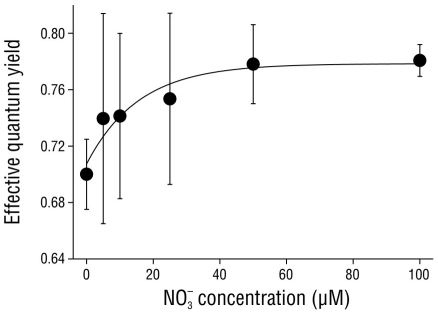
Figure 3 Effective quantum yield in Cymodocea nodosa shoots incubated with different nitrate (NO3 -) concentrations. Data points represent the average of 10 samples ± SD.
The effective quantum yield in nitrate-replete C. nodosa shoots fluctuated as a result of the irradiance level throughout the day (Fig. 4). Irradiance followed a typical sinusoidal curve, although slight deviations from the model were observed due to clear skies. The effective quantum yield in the shoots significantly decreased (P < 0.05) as irradiance increased, with minimum values observed at solar noon or 2 h afterward. However, the decrease in ΦPSII values was 4-fold greater in shoots incubated with full solar radiation (100% Eo) compared to those incubated with only 20% Eo. The ΦPSII values recovered 2 h after sundown in shoots incubated under 20%, 33%, and 60% Eo. In contrast, the ΦPSII values of shoots incubated with full solar radiation (100% Eo) did not completely recover over the same time period. Nonetheless, the ΦPSII values in these shoots completely recovered by the following morning (data not shown).

Figure 4 Incident photosynthetically active radiation (PAR) in October 2004 at the study site. Effective quantum yield values in the Cymodocea nodosa shoots incubated with different incident PAR levels. Symbols represent the mean ± SD. Error bars not shown are smaller than the symbol size.
The effective quantum yield in C. nodosa shoots was differentially affected by the irradiance they received throughout the day (Fig. 5). The first effective quantum yield value (irradiance = 0 µmol photon·m-2·s-1) corresponds to Fv/Fm, as plants were maintained in darkness throughout the night. As expected, the Fv/Fm values were greater than the ΦPSII values. In all cases, a linear decrease (P < 0.05) in ΦPSII values was observed from sunrise to solar noon. The recovery of ΦPSII values was also linear, and the same rate was observed in shoots incubated with 20% and 33% Eo (Fig. 5a, b). The slope for the recovery curve significantly decreased in the afternoon samples incubated with 60% and 100% Eo (Fig. 5c, d).

Figure 5 Effective quantum yield in Cymodocea nodosa shoots incubated with 20% (a), 33% (b), 60% (c), and 100% (d) incident irradiance (Eo). Empty symbols indicate samples from sunrise to solar noon, while filled symbols indicate samples from solar noon to sundown. Symbols represent the mean ± SD. Error bars not shown are smaller than the symbol size.
The effective quantum yield values in C. nodosa shoots under light attenuation conditions (33% Eo) fluctuated as a result of the nitrate concentration in the pre-incubation treatment (Fig. 6). Irradiance followed a typical sinusoidal curve, although dispersion of the data was observed as a result of cloud cover at the study site. In general, the ΦPSII values decreased (P < 0.05) as irradiance increased throughout the day. However, the ΦPSII values decreased to almost zero in shoots pre-incubated with no nitrate (0 µM NO3 -), whereas the ΦPSII values in shoots pre incubated with 25 and 100 µM NO3 - decreased by approximately 25% of their initial values. The effective quantum yield in shoots pre-incubated with 5 µM NO3 - decreased to values that were slightly lower than those in shoots pre-incubated with 25 and 100 µM NO3 -. The complete recovery of ΦPSII values 3 h after sundown was only observed in shoots pre-incubated with 25 and 100 µM NO3 -. In shoots incubated with 0 and 5 µM NO3 -, the complete recovery of ΦPSII values was not observed until the following morning (data not shown).

Figure 6 Incident photosynthetically active radiation (PAR) in October 2004 at the study site. Effective quantum yield values in Cymodocea nodosa shoots incubated with different incident PAR levels. Symbols represent the mean ± SD. Error bars not shown are smaller than symbol size.
The effective quantum yield values decreased as irradiance increased. The effective quantum yield before sunrise (irradiance = 0 µmol photon·m-2·s-1) corresponded to Fv/Fm, as plants were maintained in darkness throughout the night. The decrease of the effective quantum yield as a function of irradiance in the morning was significantly greater (P < 0.05) in shoots incubated with 0 and 5 µM NO3 - and lower in shoots incubated with 25 and 100 µM NO3 - (Fig. 7). In contrast, the recovery rate was similar among all treatments.
DISCUSSION
The external nitrogen concentration in the media appears to play a critical role in determining the carbon and nitrogen concentrations in C. nodosa tissues. Moreover, changes in the bio-optical characteristics of the shoots were associated with the nitrate concentration in the media, which suggests that nitrogen may be allocated in response to the light requirements of photosynthesis. Both the nitrogen and carbon content in marine algae cells have been found to rapidly change over hours to days as a result of fluctuations in the nitrate concentration in seawater (Young and Beardall 2003). As has been observed in other seaweeds and higher-plant species, a decrease in transmittance was observed as the nitrogen levels in the tissues increased in this study (Carter and Spiering 2002), which was likely the result of increasing chlorophyll levels within the tissue cells (Cabello-Pasini and Figueroa 2005). Studies conducted in several Mediterranean seagrasses have found that light relationships are similar to those found in marine macroalgae, namely, that light absorption increases non-linearly as the chlorophyll a content increases (Enriquez et al. 1994). This has implications for growth and the allocation of nitrogen to chlorophyll. For example, subtidal populations of Halophyla ovalis have shown higher tissue nitrogen content than those of intertidal plants as a direct result of elevated chlorophyll content and consequently the elevated light harvesting capacity of this species at low irradiance levels (Dawson and Dennison 1996).
In addition to irradiance, nitrate availability affected ΦPSII. Few studies have examined the effects of nutrients on the primary photochemical reactions. If nitrogen pools are allocated to chlorophyll, then limitation will directly affect the light harvesting complex. Under conditions of nitrogen limitation, the functional absorption cross-section of PSII increases along with a subsequent decrease in energy transfer between the PSII reactions centers (Young and Beardall 2003). In our study, increasing nitrate concentrations were related only to a slight increase in photosynthesis, which was measured in terms of effective quantum yield. In fact, at 0 µM NO3 - the shoots still exhibited high ΦPSII values, which may indicate that nitrogen limitation does not stimulate non-photochemical quenching (i.e., thermal dissipation) in the short-term. Alternatively, nitrogen pools may be remobilized to provide chlorophyll precursors.
The effective quantum yield of in vivo chlorophyll a fluorescence in C. nodosa varied throughout the day. This pattern has been commonly observed in macroalgae (Franklin and Forster 1997, Hader and Figueroa 1997, Hanelt 1998) and in some seagrasses, such as Posidonia oceanica (Figueroa et al. 2002). As expected, Fv/Fm at dawn (i.e., after incubation in darkness during the night) was higher than the effective quantum yield under light conditions. Enhanced thermal energy dissipation at noon has been proposed to be responsible for these diurnal variations. However, our results demonstrate that both the carbon and nitrogen content in C. nodosa tissues depend on the nitrate pre-incubation history. Nitrate availability in the media also affected the effective quantum yield determined by fluorometry. Overall, this study suggests that reductions in the nitrate concentration in seawater reduce the dynamic photoinhibition capacity of C. nodosa, which is likely to be the case for other marine macrophytes. Thus, the impacts of nutrient availability as well as nitrate in seawater on photosynthesis, respiration, and optimum quantum yield relationships require further study.